
Research Process
Planning for Research
Formulating a research proposal.
A research proposal is a simply a structured, formal document that explains what you plan to research (i.e. your research topic and question), why it’s worth researching (i.e. your justification), and how you plan to investigate it (i.e. your research approach - research and sampling methods).
Managing Resources
Managing resources is essential for any research project. Time management is essential. Examples of items that may be useful for filing and recording include a display folder, a small exercise book (for your IRP diary), a USB drive and document storage folders. Another option is to record information on smart phone or tablet apps. As you acquire information, add it into the appropriate file or folder immediately to ensure sources of information aren’t lost. Whichever system you choose, remember to back up your information in case of a computer or app crash.
Your research project is dependant on how well you manage the resources you access.
Conducting Research
Accessing sources of data.
Sources of data are imperative to your research. Individuals and Groups / Print and digital are the sources of primary or secondary data that must be assessed in order to choose the most appropriate sources of data for the topic.
Your first step should be to search secondary data for what has already been written about your chosen topic. Look at both digital and print sources of data. Your primary data will primarily come from individuals and groups, however you must choose sample groups that allow for reliable and valid research.
Example: The sources of data you chose for your topic.
Collecting and recording Data
No matter what forms of secondary data you use, the most important source of data for a research project is primary data . Primary data may include interview, survey or questionnaire responses, and observation details, from individuals, organisations or groups.
Your research methods will form the foundation of your primary data. They need to be prepared, distributed and collected in a logical, thoughtful and systematic manner to be most effective . You must think carefully about the sample method and sample size depending on your research topic.
Example: The research method and sample method you chose and how you recorded that data.
Documenting Actions and Issues
Documenting progress and issues is an important part of research i n order to learn and propose ways to improve research in the future. This is usually done through a project diary , which is a record of the steps taken during the development of your research. Your diary also includes the problems that arise and way to overcome each issue. This allows you to reflect on the research process throughout the research.
Example: The diary you kept that helped you reflect and overcome issues.
Interpreting Research
Presenting research findings.
There are two basic ways that data can be presented - Quantitative or Qualitative.
Quantitative data is in a numerical form, so it can be can be counted and then presented through the use of bar, line or pie graphs; or tables with statistics or percentages. This allows trends to be clearly seen and easily compared.
Qualitative data , such as interview data, is descriptive and often cannot be reduced to numerical form. It is often presented as a written report that describes the main ideas and trends that emerged from the research, and it includes quotes and anecdotes.
Example: How you presented data in your IRP.
Analyse Research Results
All data should be included in an analysis of your results. Before you can interpret your research findings, you need to analyse them . This means that you describe what you can see in the evidence provided in your graphs or tables . This is done by identifying the trends that you can see in your presented data
Example: How you analysed and compared data (Graphs and questionnaire responses) in your IRP.
Drawing Conclusions from Research
Drawing conclusions from your research is an extremely important part of the process. These conclusions are in relation to your initial research question, problem statement or hypothesis . This may indicate that the hypothesis upon which you have conducted your research has been proven correct OR incorrect. A good conclusion includes what your data shows, how this relates to the research in general, the validity of your findings and possible implications and recommendations from your findings
Example: How you draw conclusions from your research - Did it prove or disprove the hypothesis?
Advertisement
Diagnosis and Management of Congenital Coronary Artery Fistulas in Adults
- Congenital Heart Disease (RA Krasuski and G Fleming, Section Editors)
- Published: 11 March 2024
- Volume 26 , pages 373–379, ( 2024 )
Cite this article
- Jaya Kanduri 1 ,
- Zachary Falk 1 &
- Harsimran S. Singh 1
320 Accesses
Explore all metrics
Purpose of Review
This review describes the presentation, diagnosis, and management of congenital coronary artery fistulas (CAFs) in adults.
Recent Findings
CAFs are classified as coronary-cameral or coronary arteriovenous fistulas. Fistulous connections at the distal coronary bed are more likely to be aneurysmal with higher risk of thrombosis and myocardial infarction (MI). Medium-to-large or symptomatic CAFs can manifest as ischemia, heart failure, and arrhythmias. CAF closure is recommended when there are attributable symptoms or evidence of adverse coronary remodeling. Closure is usually achievable using transcatheter techniques, though large fistulas may require surgical ligation with bypass. Given their anatomic complexity, cardiac CT with multiplanar 3-D reconstruction can enhance procedural planning of CAF closure. Antiplatelet and anticoagulation are essential therapies in CAF management.
CAFs are rare cardiac anomalies with variable presentations and complex anatomy. CAF management strategies include indefinite medical therapy, percutaneous or surgical CAF closure, and lifelong patient surveillance.
This is a preview of subscription content, log in via an institution to check access.
Access this article
Subscribe and save.
- Get 10 units per month
- Download Article/Chapter or eBook
- 1 Unit = 1 Article or 1 Chapter
- Cancel anytime
Price includes VAT (Russian Federation)
Instant access to the full article PDF.
Rent this article via DeepDyve
Institutional subscriptions
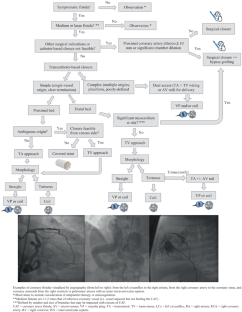
Similar content being viewed by others
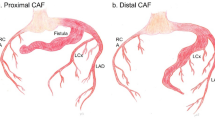
Diagnosis and Management of Congenital Coronary Artery Fistulas in Infants and Children
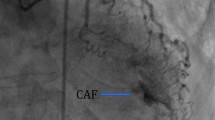
Coronary artery fistulae

Long-Term Follow-Up of Pediatric Patients After Congenital Coronary Artery Fistula Closure
Explore related subjects.
- Medical Imaging
Data Availability
The authors confirm that the data supporting the findings of this review are available within the article.
Papers of particular interest, published recently, have been highlighted as: • Of importance •• Of major importance
Rao SS, Agasthi P. Coronary artery fistula. StatPearls. 2023 June 5; https://www.ncbi.nlm.nih.gov/books/NBK559191/
Yun G, Nam TH, Chun EJ. Coronary artery fistulas: pathophysiology, imaging findings, and management. Radiographics. 2018;38(3):688–703.
Article PubMed Google Scholar
• Al-Hijji M, El Sabbagh A, El Hajj S, AlKhouli M, El Sabawi B, Cabalka A, et al. Coronary artery fistulas: indications, techniques, outcomes, and complications of transcatheter fistula closure. JACC: Cardiovasc Interv. 2021 July 12; 14(13):1393–406. This study highlights contemporary procedural techniques of transcatheter coronary artery fistula closure, as well as indications, outcomes, and complications of this procedure.
Brantner P, Madaffari A, Fahrni G, Zellweger MJ, Haaf P. 3D-printed visualization of a complex coronary-venous fistula with additional feeders from the descending aorta. J Am Coll Cardiol Case Rep. 2020;2(11):1736–8.
Google Scholar
• Kanduri J, Truong QA, Wong SC, Bergman G, Kim L, Holzer R, et al. Percutaneous closure of giant aneurysmal coronary artery-to-coronary sinus fistulae with guidance from three-dimensional printed models: a case series. Eur Heart J Case Rep. 2023 Jan 10; 7:1–6. This case series highlights the use of computed tomography to generate 3D reconstructed models to help augment visuospatial understanding of coronary artery fistula, and to facilitate manual bench testing of devices for closure.
Warnes CA, Williams RG, Bashore TM, Child JS, Connolly HM, Dearani JA, et al. ACC/AHA 2008 Guidelines for the management of adults with congenital heart disease: executive summary. Circulation. 2008;118(23):2395–451.
Hobbs RE, Millit HD, Raghavan PV, Moodie DS, Sheldon WC. Coronary artery fistulae: a 10-year review. Cleveland Clinic Quarterly. 1982 Winter; 49(4):191–7.
Sunder S, Balakrishnan KG, Tharakan JA, Titus T, Pillai VR, Francis B, et al. Coronary artery fistula in children and adults: a review of 25 cases with long-term observations. Int J Cardiol. 1997;58(1):47–53.
Article CAS PubMed Google Scholar
Davis JT, Allen HD, Whellar JJ, Chan DP, Cohen DM, Teske DW, et al. Coronary artery fistula in the pediatric age group: a 19-year institutional experience. Ann Thorac Surg. 1994;58(3):760–3.
Download references
Author information
Authors and affiliations.
Division of Cardiology, Department of Medicine, Weill Cornell Medical College, New York Presbyterian Hospital, 520 East 70 Street, New York, NY, 10021, USA
Jaya Kanduri, Zachary Falk & Harsimran S. Singh
You can also search for this author in PubMed Google Scholar
Contributions
J.K. and H.S. wrote the main manuscript text and Z.F. prepared the figures and tables. All authors reviewed the manuscript.
Corresponding author
Correspondence to Jaya Kanduri .
Ethics declarations
Conflict of interest.
The authors declare no competing interests.
Human and Animal Rights and Informed Consent
This article does not contain any studies with human or animal subjects performed by any of the authors.
Additional information
Publisher's note.
Springer Nature remains neutral with regard to jurisdictional claims in published maps and institutional affiliations.
Rights and permissions
Springer Nature or its licensor (e.g. a society or other partner) holds exclusive rights to this article under a publishing agreement with the author(s) or other rightsholder(s); author self-archiving of the accepted manuscript version of this article is solely governed by the terms of such publishing agreement and applicable law.
Reprints and permissions
About this article
Kanduri, J., Falk, Z. & Singh, H.S. Diagnosis and Management of Congenital Coronary Artery Fistulas in Adults. Curr Cardiol Rep 26 , 373–379 (2024). https://doi.org/10.1007/s11886-024-02038-1
Download citation
Accepted : 01 March 2024
Published : 11 March 2024
Issue Date : May 2024
DOI : https://doi.org/10.1007/s11886-024-02038-1
Share this article
Anyone you share the following link with will be able to read this content:
Sorry, a shareable link is not currently available for this article.
Provided by the Springer Nature SharedIt content-sharing initiative
- Coronary artery fistulas
- Transcatheter closure
- 3-D reconstructed models
- Find a journal
- Publish with us
- Track your research
CAFS - RESEARCH METHODOLOGY

Students also studied

-Sample method -Sample group -Sample size Choose matching term 1 What is the entire research process? 2 Define hypothesis 3 What are the considerations that must be made during sampling? 4 What is the purpose of research? Don't know?
- Open access
- Published: 02 October 2023
Define cancer-associated fibroblasts (CAFs) in the tumor microenvironment: new opportunities in cancer immunotherapy and advances in clinical trials
- Hao Zhang 1 ,
- Xinghai Yue 1 , 2 na1 ,
- Zhe Chen 1 ,
- Chao Liu 3 ,
- Wantao Wu 4 ,
- Nan Zhang 5 ,
- Zaoqu Liu 6 ,
- Liping Yang 7 ,
- Qing Jiang 2 ,
- Quan Cheng 8 , 9 ,
- Peng Luo 1 , 10 &
- Guodong Liu 1
Molecular Cancer volume 22 , Article number: 159 ( 2023 ) Cite this article
13k Accesses
46 Citations
7 Altmetric
Metrics details
Despite centuries since the discovery and study of cancer, cancer is still a lethal and intractable health issue worldwide. Cancer-associated fibroblasts (CAFs) have gained much attention as a pivotal component of the tumor microenvironment. The versatility and sophisticated mechanisms of CAFs in facilitating cancer progression have been elucidated extensively, including promoting cancer angiogenesis and metastasis, inducing drug resistance, reshaping the extracellular matrix, and developing an immunosuppressive microenvironment. Owing to their robust tumor-promoting function, CAFs are considered a promising target for oncotherapy. However, CAFs are a highly heterogeneous group of cells. Some subpopulations exert an inhibitory role in tumor growth, which implies that CAF-targeting approaches must be more precise and individualized. This review comprehensively summarize the origin, phenotypical, and functional heterogeneity of CAFs. More importantly, we underscore advances in strategies and clinical trials to target CAF in various cancers, and we also summarize progressions of CAF in cancer immunotherapy.
Introduction
The tumor microenvironment (TME) has been studied in depth with the progression of research on solid tumors. TME refers to the surrounding microenvironment tumor cells reside and develop, including surrounding blood vessels, the extracellular matrix (ECM), multiple signaling molecules, and non-neoplastic cells like immune cells, fibroblasts, lipocytes, etc. [ 1 , 2 ]. Among all those various TME components, cancer-associated fibroblasts (CAFs) have been noted to exhibit a higher correlation with tumor development and have become a hot spot for oncology research [ 3 ].
CAFs are widely known for their significant heterogeneity, which is reflected explicitly in the substantial subpopulation of CAFs [ 4 ], as well as the juxtaposition of tumor-promoting and tumor-restraining [ 5 ]. As a substantial component of tumor stroma, CAFs perform an essential function in tumor progression and metastasis [ 6 ], including ECM depositing and remodeling [ 7 ], having crosstalk with immune cells [ 8 ], promoting cancer cell proliferation, angiogenesis, and drug resistance [ 9 , 10 , 11 ]. Simultaneously, some research indicates that CAFs could exert tumor-restraining functions in particular cancer types [ 12 , 13 ].
Studies of interaction with TME identified numerous mechanisms, thus presenting multiple potential targets for oncotherapy. Nevertheless, various clinical trials of treatment strategies targeting CAFs have failed and, in some cases, even culminated in accelerating cancer progression and resulting in shortened patient survival [ 5 ]. The reasons for this are that the role of CAFs in tumorigenesis and development has not been fully elucidated, and that the function of CAFs is context-dependent and has significant plasticity. Therefore, more research is urgently needed to investigate the potential of CAFs as therapeutic targets for oncotherapy.
This review will initially summarize the background knowledge of CAFs, especially their heterogeneity and the pro-tumor functions of CAFs, including angiogenesis, metastasis, extracellular matrix remodeling, immunosuppression, etc. We will also introduce the current status of research on CAF as a potential tumor therapeutic target. Finally, we will present the latest advances in oncology therapeutic research and clinical trials for CAF in several cancer types.
Background knowledge of CAFs
Overview of tme.
With the deepening of cancer research, increasing evidence continuously proves that TME is closely pertaining to nearly all stages of cancer, and the existing research model has gradually changed from tumor-centric to TME-centric. TME is typically defined as a multicellular niche characterized by a hypoxic, acidic environment. The main cellular components include immune cells such as T and B lymphocytes, macrophages, dendritic cells (DC), natural killer (NK) cells, and myeloid-derived suppressor cells (MDSC); stromal cells like CAFs, pericytes, and mesenchymal stromal cells; other non-cellular components like ECM, blood vessels, lymphoid organs or lymph nodes, nerves, and chemokines. The classification of T cells is complex and will not be described in detail here. T cells in TME mainly include CD4 + T cells, CD8 + T cells, Tregs, and γδ T cells. CD8 + T cells are robust effector cells that release granzyme and perforin-induced apoptosis in tumor cells. CD4 + T cells are helper T cells, divided into th1 and th2 types, and their effects are also opposed. Treg is the key to maintaining immune balance in the body and mainly plays an anti-tumor role in TME. γδ T cells are a specialized subset of T cells that express γδTCR and recognize target antigens in an MHC-independent manner. γδT cells also play a dual role, secreting IL-17 to inhibit the anti-tumor immune response and also exerting cytotoxic effects to kill tumor cells [ 14 , 15 , 16 , 17 , 18 ]. B cells are another large class of specific immune cells, majorly involved in humoral immunity. The dual effect of B cells on tumors is manifested by secreting pro-inflammatory factors, activating complement to suppress immunity, and directly killing tumor cells [ 19 ]. As for macrophages, they are divided into two subgroups, M1 has antitumor effects, and M2, on the contrary, promotes tumor development by suppressing immunity, promoting angiogenesis and metastasis. T cells, B cells, and antigen-presenting cells are collectively called specific immune cells, of which dendritic cells are a type of antigen presenting cell (APC) that integrates information from TME and transmits it to other immune cells [ 20 ]. Mast cells are a type of granulocytes that play an important role in type 1 hypersensitivity and autoimmunity, and they can secrete cytokines in TME that promote angiogenesis, tumor invasion, and kill tumor cells [ 21 ]. NK cells are non-specific immune cells that can kill tumor cells in a variety of ways, hence they have a strong anti-cancer ability. But tumor cells can escape by, for example, inhibiting the upregulation of receptors [ 22 ]. Moreover, monocytes are precursors of macrophages and dendritic cells, and neutrophils, eosinophils, and basophils are all granular leukocytes, which also have dual functions of anti-tumor and tumor suppression [ 23 , 24 , 25 , 26 ]. Mesenchymal stromal cells are derived from the mesoderm in early development, with self-replication ability and multidirectional differentiation potential. It secretes TGF-β and other chemical factors in TME to promote tumor progression and also has tumor cytotoxicity to inhibit tumor growth. Myeloid-derived suppressor cells, composed of immature monocytes and neutrophils, can inhibit the function of a wide range of immune cells and are therefore associated with poor clinical outcomes [ 27 , 28 ]. Pericytes are adjacent to endothelial cells, and they have been reported to be associated with TME immunosuppressive states and epithelial-to-mesenchymal transition (EMT). Lastly, adipocytes are closely related to cancer cells. They release free fatty acids, hormones, cytokines, adikines, and growth factors that affect cancer cells and host cells in TME [ 29 , 30 ].
These biological constituents do not function independently but interact to influence tumor progression by secreting various chemical factors, chemokines, exosomes, etc. Briefly, the cellular composition and functional status of TME varies depending on a range of conditions such as the site of tumorigenesis, the inherent characteristics of cancer cells, tumor stage, and patient features. Alterations in TME are inseparable from crosstalk between tumor cells and cellular components within TME. As one of the most abundant cell types in TME, CAF is the center of cross-communication among various cells in the tumor stroma. Analogous to most of the abovementioned cells, the fact that CAFs display both pro-tumor and anti-tumor functions within TME is not unexpected. The tumor-promoting function of CAF is multifaceted, such as participating in the reconstruction of ECM and the formation of the immunosuppressive microenvironment, but its specific subtype has also been observed to have tumor suppressive function, which will be described in more detail later [ 31 , 32 , 33 ]. The functional differences between CAF and other cells within TME are roughly summarized in Table 1 [ 14 , 15 , 16 , 17 , 19 , 20 , 21 , 22 , 23 , 24 , 25 , 26 , 27 , 28 , 29 , 30 , 34 , 35 , 36 , 37 , 38 , 39 , 40 , 41 , 42 , 43 , 44 , 45 , 46 , 47 , 48 , 49 , 50 , 51 , 52 ].
Biomarkers, origins and regulation of CAFs
Biomarkers of cafs.
Generally, fibroblasts are defined as interstitial cells of the mesenchymal lineage. Fibroblasts are cells that produce collagen and contribute to the formation of connective tissues, which help maintain the typical structure of tissues. Quiescent fibroblasts are activated during wound healing and neoplasia. As a result, the currently widely accepted hypothesis is that CAFs are activated by fibroblasts located in or near tumors in the context of tumors, which is also why they are called CAFs [ 3 , 5 , 53 ].
Several different biomarkers are used to define CAFs, including but not limited to α-smooth muscle actin (α-SMA, also known as ACTC2), platelet-derived growth factor receptorα/β (PDGFRα/β), fibroblast-specific protein 1 (FSP-1, also known as S100A4), fibroblast activation protein (FAP) [ 54 , 55 , 56 , 57 ]. Nevertheless, the specific biomarker that can define all sorts of CAFs has not been found yet. Among these biomarkers, FAP, a type II transmembrane glycoprotein, whose expression was selectively observed in CAFs and pericytes in most human epithelial cancers, was thought to facilitate tumor growth and proliferation [ 58 , 59 ]. To date, it is extensively considered to be the most promising target of CAF-based oncotherapy [ 58 , 60 , 61 ]. Depletion of FAP-positive fibroblasts caused necrosis of both tumor and stroma cells in a transgenic mouse model of lung cancer [ 62 ], which reflected the tumor-promoting function of FAP from another aspect. More information on the progress of the treatment will be provided in detail later.
Origins of CAFs
As a result of being devoid of specific biomarkers to define all CAFs, the precise cellular origin of CAFs still needs to be fully elucidated. Lineage tracing studies showed many putative cellular precursors [ 49 , 63 ]. CAFs can derive from resident fibroblasts [ 64 ]. These resident fibroblasts, quiescent pancreatic stellate cells (PSCs), and hepatic stellate cells (HSCs) can acquire a myofibroblast-like phenotype in the liver and pancreas, including α-SMA expression. In that case, these two cells are considered CAFs in pancreatic and liver cancers, respectively [ 65 , 66 ]. Despite the local cellular sources, CAFs can also originate from bone marrow-derived mesenchymal stem cells (BM-MSCs) [ 27 ], which is confirmed by both in vitro and in vivo tracing studies [ 67 , 68 , 69 ]. Epithelial and endothelial cells were reported to adopt a fibroblastic phenotype with the expression of S100A4 through EMT and endothelial-to-mesenchymal transition (EndMT), respectively, making them a possible origin of CAFs [ 56 , 70 ]. The expression of CAF- and EMT-related markers and proteins has also been highly correlated with the progression of skin malignancies [ 71 ]. Moreover, recent studies have demonstrated in non-small cell lung cancer (NSCLC) that CAFs can derive from macrophage via macrophage-myofibroblast transition (MMT), which is also relevant to fibrotic nephropathy [ 72 , 73 ]. Except for the aforementioned familiar sources, other possible sources include pericytes [ 74 ] and adipocytes [ 75 ] (Fig. 1 ).
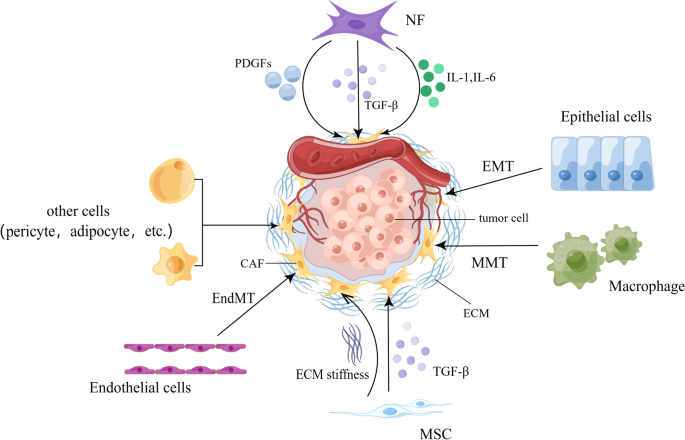
Possible origins of CAF. ECM, extracellular matrix; EMT, epithelial-to-mesenchymal transition; EndMT, endothelial-to-mesenchymal transition; IL, interleukin; MMT, macrophage-to-mesenchymal transition; MSC, mesenchymal stem cells; NF, normal fibroblast; PDGF, platelet-derived growth factor; TGF-β, transforming growth factor beta. By Figdraw
Regulation of CAFs
In particular, researchers have concentrated on dissecting the modification process of NF (normal fibroblast)-CAF transition for a long time. It is believed that the genome of CAF is relatively stable, and transcriptional regulation plays an instrumental role in reprogramming. Lee, K.-W. et al . demonstrated the existence of a master transcription factor (mTF) PRRX1 in vivo and in vitro, which closely pertained to fibroblast acquisition of the CAF phenotype. Transcription factor SOX2 was revealed to participate in this process as well [ 76 , 77 , 78 ]. DNA methylation and histone methylation/acetylation are two major alterations within epigenetic modifications that affect the transcriptional factors. In cancer cells, the presence of global DNA hypomethylation and local DNA hypermethylation were both observed, and similar patterns were found in CAFs by numerous research. Recent investigations have shown that CAF DNA methylation depends on the kind of cancer, with some CAFs having abnormal, not just reduced, methylation, even if the trend of global DNA hypomethylation persists in CAFs from many malignancies. Moreover, recent research has emphasized the significance of histone methylation for CAF function. For instance, during enhancer reprogramming, the histone acetylation and methylation mark histone H3 lysine 27 acetylation (H3K27ac) and histone H3 lysine 4 mono-methylation (H3K4me1) was found, accompanied with CAF activation [ 79 ]. The loss of S-adenosyl methionine-mediated histone hypomethylation caused nicotinamide N-methyltransferase (NNMT) production in CAFs to enhance cytokine secretion and ECM deposition in ovarian cancer. Additionally, CAFs contain several mediators of epigenetic control. TGF-β, LIF, JAK1/STAT3, IL-1, IL-1, TNF-α, IL-6, and HIF-1 are well-known soluble factors that activate CAF. The surrounding TME drives the change from NFs to CAFs during CAF maturation. The miRNAs' ability to contribute to and adapt to the surrounding milieu has led to their involvement in this transient process. Examples of these miRNAs are miR-149, miR-27a, miR-29a-3p, miR-30c-5p, and miR-200 s/miR-221 [ 78 , 80 ].
Heterogeneity of CAFs
Cellular phenotype heterogeneity of cafs.
Studies of human cancers and mouse models using immunostaining, in situ hybridization, flow cytometry, fluorescence-activated cell sorting, and mRNA microarrays validated the existence of distinct CAF subsets. More recently, thanks to the emergence and application of single-cell RNA sequencing (scRNA-seq), cellular heterogeneity has been detected, improving the resolution of gene expression studies, which enables a deeper understanding of CAF subsets in different tumor types [ 4 ]. By analyzing a wide range of biomarkers selectively expressed on the surface of CAFs in specific TMEs, numerous CAF phenotypes have been defined in different cancers, reflecting the significant phenotypical heterogeneity of the CAFs [ 81 , 82 , 83 , 84 , 85 , 86 , 87 , 88 , 89 , 90 , 91 , 92 , 93 , 94 ].
In pancreatic ductal adenocarcinoma (PDAC), Öhlund and colleagues distinguished two distinct CAF subpopulations, inflammatory CAFs (iCAFs) and myofibroblastic CAFs (myCAFs). iCAFs exhibited low expression of αSMA and high expression of cytokines such as IL6, IL11, and PDGFRα [ 81 ]. Moreover, iCAFs were reported to be induced by circCUL2/ microRNA (miR) -203a-5p/NF-κB/IL6 axis from NFs [ 95 ]. In contrast, FAP + myCAFs had a selectively high expression of αSMA and lacked expression of inflammatory cytokines. The spatial distribution discrepancy of these two CAF subsets was observed via immunostaining of tumor organoids. Specifically, myCAFs were located near neoplastic cells, whereas iCAFs were more distant [ 81 ]. Despite PDAC, these two CAF subpopulations were also defined in other cancer contexts [ 94 , 96 ]. In another study, Elyada et al. identified ‘antigen-presenting CAFs (apCAFs)’, which expresses Major Histocompatibility Class (MHC) II and CD74 but no classic costimulatory molecules (CD80, CD86, CD40), in KPC tumors by using scRNA-seq and immunohistochemical analysis. Researchers revealed that apCAFs were able to activate CD4 + T cells in an antigen-specific fashion, confirming their putative immune-modulatory capacity [ 92 ]. Another scRNA-seq of fibroblasts from different stages of KIC tumors found that mesothelial cells in the normal pancreas had a similar genetic profile to apCAFs, suggesting that apCAFs may originate from mesothelial cells [ 97 ].
Concomitant analyses of six biomarkers, including FAP, CD29 (integrin-β1), α-SMA, FSP1, PDGFRβ, and caveolin, characterized four CAF subsets (from CAF-S1 to CAF-S4) with distinct properties in ovarian and breast cancers (BC) [ 94 , 98 ]. Kieffer et al. further identified eight different CAF-S1 clusters (from cluster 0 to cluster 7) in BC by using scRNA-seq. Two of these CAF -S1 clusters, namely ECM-myCAF and TGFβ-myCAF, were found to play an imperative role in forming an immunosuppressive environment and resisting immunotherapy. ECM-myCAF was demonstrated to stimulate the expression of both PD-1 and CTLA-4 protein at the surface of CD4 + CD25 + T lymphocytes, and PD-1 + CTLA4 + Tregs can reciprocally alter the proportion of TGFβ-myCAF through converting ECM-myCAF into TGFβ-myCAF [ 93 ]. In 2018, researchers observed that CAFs in the MMTV-PyMT mouse model of BC can be classified into four distinct categories: vascular CAFs (vCAFs), matrix CAFs (mCAFs), cycling CAFs (cCAFs), and developmental CAFs (dCAFs). Among them, vCAF was derived from the perivascular area and mCAFs originated from resident fibroblasts, and these subsets were also of different clinical significance [ 83 ]. Ds, F. et al . identified six transcriptionally distinct clusters of CAFs in endogenous mouse breast tumors. They further signified three major clusters using spatial transcriptomics, which were mechanoresponsive (MR) CAF, steady state-like (SSL) CAF, and immunomodulatory (IM) CAF, and these subpopulations were found conservative across multiple solid tumor tissues and species [ 86 ]. More recently, single-cell transcriptomics revealed that CAF in BC originates from CD26 + and CD26 − NF populations, and then they differentiated into specific functional subpopulations [ 99 ].
The application of scRNA-seq in human gastric cancer (GC) has identified a prior undetected subset of CAF, characterized by high expression of Periostin (POSTN), which encodes a protein that functions as an adhesion-modulating factor in the ECM component. This CAF subpopulation is highly expressed in genes involved in ECM remodeling and is therefore defined as extracellular matrix CAFs (eCAFs). Furthermore, tumor-derived eCAFs, as important components in TME to promote metastasis, are inseparable from the increase in gene expression associated with tumor invasion. Simultaneously, high POSTN expression is associated with GC patients’ unsatisfactory overall survival (OS), demonstrating its potential value in predicting prognosis [ 96 ]. Lambrechts and colleagues defined seven subsets of fibroblasts by scRNA-seq analysis of stromal cells derived from excised NSCLC tumor tissue and non-tumor lung tissue. They identified five types of fibroblasts in cancerous tissue and detected marker genes for each subpopulation [ 88 ]. Lastly, a study conducted by Galbo, P. M. and colleagues identified six CAF subtypes that are generally observed in melanoma, head and neck squamous cell carcinoma, and lung cancer. Specific subpopulations including pan-myCAF, pan-dCAF, pan-iCAF, pan-pCAF, and pan-iCAF-2 were found pertaining to immunotherapy resistance [ 91 ]. More information about CAF phenotypic heterogeneity is summarized in Tables 2 .
Currently, defining functional populations of CAFs using cell surface biomarkers is still a challenging task. Because the cell source of CAF is not monolithic, it is almost impossible to identify universal CAF markers across different cancer types. Future studies could combine scRNA-seq and in vivo models to better elucidate the heterogeneity of CAF in the context of cell origin, surface markers, RNA profiles, activation phases, and spatial distribution.
Functional heterogeneity of CAFs
As one of the major components of TME, CAFs have been shown to interact with tumors by multiple mechanisms: inducing tumor cell proliferation [ 9 ], affecting tumor angiogenesis [ 10 ], shaping immunosuppressive microenvironment to escape from immune surveillance [ 100 ], and promoting tumor formation and drug resistance [ 11 ]. For the above-mentioned reasons, CAFs are historically considered imperative tumor-promoting components. However, as a result of intensive research, much evidence supporting the tumor-inhibitory effects of CAFs has emerged, suggesting that the role of CAFs is not singularly promotional or inhibitory, but rather falls somewhere in the middle. For instance, the depletion of αSMA + myofibroblasts in PDAC suppressed tumor immune surveillance with an increase in the percentage of regulator T cells (Treg, CD4 + Foxp3 + ), which led to aggressive tumor progression and reduced animal survival [ 101 ]. In addition, Bhattacharjee et al. discovered that myCAF-expressed type I collagen had a tumor-restraining role in PDAC and colorectal cancer (CRC) metastasizing to the liver, it suppressed tumor growth by mechanically restraining tumor spread [ 13 ]. Consistent with the previously mentioned, Chen et al. deleted type I collagen in αSMA + myofibroblasts in pancreatic cancer (PC) mouse model, significantly reducing the OS of mice and accelerating PDAC progression [ 102 ]. These studies demonstrate that some CAF subpopulations have tumor suppressor effects to some extent. On the basis of current findings, CAFs can be described as a group of cells with functional heterogeneity (Fig. 2 ). Research and work are urgently entailed to elucidate the clinical relevance of CAF heterogeneity. Below, we will elaborate on the crosstalk mechanisms between CAF and tumor components.
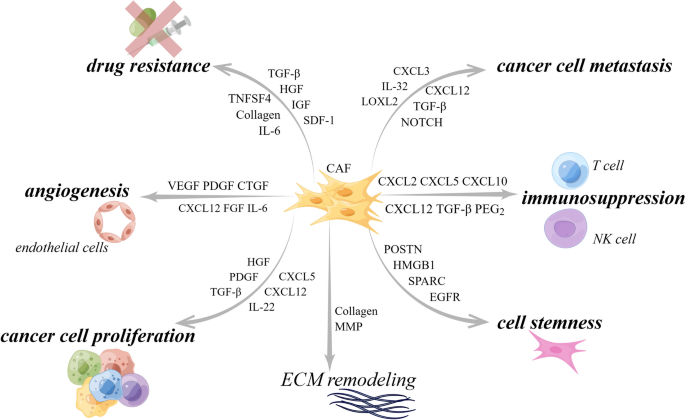
Functional heterogeneity of CAF. CAF is broadly classified as pro-tumor CAF and tumor-suppressing CAF, both of which affect tumor progression through multifaceted mechanisms. However, there are still other potential functions that have not been discovered, and it is not yet possible to determine whether this function is beneficial or harmful to tumor progression. ECM, extracellular matrix; SHH, Sonic Hedgehog. By Figdraw
The cancer-promoting functions of CAFs
Accumulating evidence continues to signify that CAFs have pleiotropic pro-tumor functions, including tumor cell proliferation, tumor angiogenesis, tumor invasion and metastasis, drug resistance, etc.
Facilitating proliferation
Persistent proliferation is one of the quintessential malignant phenotypes of cancer cells. Cancer cells can stimulate proliferation through autocrine and interact reciprocally with other cells in TME to form feedback signals to promote proliferation [ 103 ]. Among them, the cross-linking between CAF and cancer cells is extensively reported. Glucose, amino acids, lipids, etc., are the material foundation of cell proliferation. However, CAF was found to change and reprogram the behavior of metabolism of the above substances in tumor cells and directly provide nutrients to them. Intriguingly, CAF can also impact cancer metabolism through secreting exosomes. As a molecular sponge of miR-330-5p in BC cells, exosomal long noncoding RNA (lncRNA) SNHG3 can suppress mitochondrial function, expedite glycolysis, and enhance breast tumor cell proliferation [ 104 , 105 ]. Some evidence suggested that the prostaglandin E2 (PGE 2 ) pathway expressed by CAF highly correlates with the proliferative process. In the neuroblastoma xenograft model, a remarkable reduction in tumor cell proliferation was observed by immunohistochemical staining after inhibition of the PGE 2 pathway by microsomal prostaglandin E synthase-1 (mPGES-1) inhibitors [ 106 , 107 ]. It is noteworthy that PGE 2 signaling is contradictory in promoting proliferation and metastasis. Elwakeel, E. et al . observed growth inhibition of primary tumors in mice after knocking out prostanoid E receptor 3 (EP3) restriction PGE2 signaling in CAF. Still, the induction of metastatic features of tumor cells and the regulation of CAF phenotypes were also investigated [ 107 ]. CXCL12/CXCR4 cascade in FAP + CAF also contributed to cancer cell proliferation [ 108 ]. In addition, CAF-expressed methyltransferase NNMT in tumor stroma can support ovarian cancer proliferation. It becomes a potential therapeutic target because of its multifaceted metabolic regulatory functions, including cancer progression and CAF differentiation [ 109 ].
Potentiating angiogenesis
CAF has been reported to contribute to tumor angiogenesis through VEGF-dependent and VEGF-independent pathways [ 110 , 111 , 112 ]. In PDAC, scRNA-seq analysis technology has been used to confirm that CAF overexpresses several proangiogenic factors, supporting the pro-angiogenic effect of CAF [ 101 ]. CAFs produce angiogenesis regulators, such as VEGFA, PDGFC, FGF2, CXCL12, osteopontin, and CSF3 to promote the growth of tumor-associated blood vessels by recruiting myeloid cells and accelerate tumor angiogenesis by attracting vascular endothelial cells and recruiting monocytes [ 5 , 49 , 113 ]. CAF can also increase the formation of vascular mimicry (VM), and the contact between cancer cells and CAF via the Notch2-Jagged1 pathway contributes to the formation of VM networks. Simultaneously, the formation of VM was associated with anti-VEGF treatment resistance, and the combination treatment with anti-VEGF antibody and γ-secretase inhibitor DAPT, which can inhibit the Notch signaling, significantly restrained the growth of lung cancer [ 114 ]. In addition, the deletion of connective tissue growth factor (CTGF) belonging to the CCN family has been shown in melanoma studies to affect CAF activation and neovascularization, suggesting that CAF-derived CTGF is highly correlated with tumor angiogenesis. At the same time, CAFs-secreted CTGF has also been found to be associated with a poor prognosis for malignant mesothelioma, promoting metastasis [ 115 , 116 , 117 , 118 ]. Subsequently, Chitinase 3-like 1 (CHI3L1) secreted by CAFs acts on CAFs to increase IL-8 secretion and promote angiogenesis in CRC [ 119 ].
Promoting invasion and metastasis
CAFs also exert their pro-tumor function by affecting tumor metastasis. Fibronectin (Fn) is a large outer cell membrane protein found on the surface of various animal cells. Fn plays a vital role in cell adhesion, regulating cell polarity and differentiation. CAFs align Fn by increasing contractility and traction, promoting directed migration of prostate and pancreatic cancer cells, which are mediated by α5β1 integrins and PDGFRα [ 120 ]. It is well described that in BC, distinct amounts of S1 CAFs and S4 CAFs were found in metastatic breast cancer axillary lymph nodes, conducting tumor cell migration and invasion via CXCL12, TGFβ, and NOTCH signaling pathways, respectively [ 89 , 121 , 122 ]. The overexpression of RHBDF2 activated by TGFβ1 signaling can be observed in CAFs isolated from human diffuse-type gastric cancers (DGC), which can enhance the motility of CAF, and the highly active CAF, in turn, helps DGC cells to invade [ 123 ]. Moreover, Daniel, S. and colleagues indicated that CAFs promote GC cell survival and metastasis via activating CXCL12/CXCR4 axis. GC cell invasion was inhibited after CXCR4 antagonist (AMD3100) treatment, indicating that targeting CXCL12/CXCR4 might be a promising therapy in clinical treatment [ 124 , 125 ]. Hemalatha, S. K. et al . have demonstrated that the conversion process from CAF to Metastasis Associated Fibroblasts (MAFs), a type of cell associated with the metastasis process, can be mediated by cancer cells, further promoting cancer metastasis [ 126 , 127 ]. Additionally, PDAC metastasis was reported to be induced by myoCAF through type III collagen hyperplasia via the IL-33-ST2-CXCL3-CXCR2 axis. Heparan sulfate proteoglycan 2 (HSPG2) or perlecan, whose pro-metastasis function was identified, was observed more expression in metastatic CAFs than in weakly metastatic cancer. Intriguingly, primary CAFs named mutant-educated CAFs isolated from KPflC and KPC mice established a microenvironment conducive to invasion [ 128 , 129 , 130 , 131 ]. Of note, several cytokines derived from the CAF display confirm its pro-metastasis features. For instance, CXCL5, regarded as an invasive phenotype of tumor cells, can indirectly facilitate tumor growth. According to Zhou, S.-L, CXCL5 exacerbated intrahepatic cholangiocarcinoma (ICC) progression and metastasis by recruiting intratumoral neutrophils [ 5 , 132 , 133 ]. Another CAF-secreted chemokine, CCL5, can induce metastasis of hepatoma cells, which was achieved by inhibiting hypoxia-inducible factor 1α (HIF1α) degradation, thereby upregulating the gene zinc finger enhancement protein 1 (ZEB1) and inducing EMT [ 134 ]. Microfibrillar-associated protein 5 (MFAP5) was reported to facilitate the proliferation and invasion of bladder cancer cells in vivo and in vitro experiments [ 135 ]. In addition, fibroblast growth factor-2 (FGF2) was observed to promote BC cell migration and invasion through the paracrine FGF2-FGFR1 circle [ 136 ]. More importantly, CAFs-derived interleukins are essential in tumor progression and metastasis. IL-6, abundantly expressed in tumors, can protect gastric cancer cells through paracrine signaling and promote the invasion of BC cells. On this account, the hidden mechanism entails deeper exploration. What’s more, IL32 promotes the invasion and metastasis of BC cells through the integrin β3-p38 MAPK signaling pathway [ 137 ]. IL33 has been shown to facilitate lung metastasis in BC via instigating type 2 inflammation [ 138 ].
ECM is a complicated network comprising diverse extracellular-secreted macromolecules. The major compositions of ECM are glycoproteins, proteoglycans (PGs), and fibrous proteins like collagens and elastins [ 139 , 140 ]. ECM is best described as the environment in which cells can develop [ 141 ]. Normal fibroblasts are embedded in the fibrillar ECM of the interstitium and do not associate with the basement membrane [ 7 ]. Whereas CAFs play an essential role in remodeling ECM. CAFs can synthesize ECM proteins and ECM-remodeling enzymes. The magnificent ECM biosynthesis and deposition ability of CAFs makes neoplastic tissues stiffer than normal tissues. Matrix metalloproteinases (MMPs), a family of zinc-dependent endopeptidases and one of the ECM-degrading proteases, were first described by Gross and Lapiere in 1962. The production of MMPs allows CAFs to degrade the ECM, further facilitating cancer cell invasion and making MMPs viable cancer targets. In lung cancer, the increase in tumor tissue stiffness can be attributed to the remodeling of the ECM and the secretion of growth factors by CAFs, which improve the attachment of metastatic cancer cells to the tumor endothelium, thereby exacerbating the progression of metastatic tumors. Moreover, large amounts of deposited ECM can exert a protective function via upregulating programmed death-1 receptor-ligand (PD-L1) expression in lung cancer cells [ 139 , 142 , 143 , 144 ]. Nguyen, E. V. et al . revealed that CAF-secreted lysyl oxidase-like 2 (LOXL2) could expedite ECM alignment, which was conducive to the migration of prostate CAF and cancer cell [ 142 , 145 ].
Drug resistance
Multiple findings validated that CAFs can contribute to chemotherapy and radiotherapy resistance through numerous mechanisms, which led to therapeutic failure. Conversely, CAF can enhance tumor cell resistance by directly secreting cytokines and delivering exosomes. The previously mentioned CAF subtype expressing inflammatory factors in melanoma inhibited immune-checkpoint blockade (ICB) therapy response, and CAF-secreted CXCL12 contributed to tumor progression and gemcitabine resistance via upregulating SATB-1 secretion [ 91 , 146 ]. P35 was a vital cancer suppressor gene, and IL-6, secreted by CAF, has been reported to exert a protective effect on cancer cells. IL-6 attenuated the p53 response via the JAK/STAT pathway, inhibited doxorubicin-induced cell death, and increased the survival of prostate cancer cells [ 147 , 148 ]. Still, in prostate cancer, CAF-derived exosomes miR-423-5p inhibited the GREM2 (Gremlin 2) gene via the TGF-β pathway, increasing resistance to taxane. The exosome miR-22 secreted by CD63 CAFs can bind to ERα and PTEN, and confer tamoxifen resistance in BC cells. Furthermore, CD63 neutralizing antibodies counteracted these responses, suggesting that CD63 CAF may be a possible target to restore sensitivity to tamoxifen therapy [ 149 ]. Alternatively, exosome LINC00355 has been demonstrated to promote cisplatin resistance of bladder cancer cells via the miR-34b-5p/ABCB1 axis. Previous studies have shown that exosomal LINC00355 can facilitate the proliferation and invasion of bladder cancer cells as well [ 150 , 151 , 152 ]. Apart from this, Fang, Y. and colleagues found that CAF had endogenous resistance to gemcitabine compared to NF. CAF also delivered miR-106b directly to pancreatic cancer cells via exosomes, targeting the TP53INP1 gene to promote GEM resistance in cancer cells [ 153 ]. On the other hand, CAF boosted drug resistance by interacting with other TME cellular components. A study by Haldar, S. and colleagues reported that the synergistic effect of docetaxel and C3aR could impair the mitochondrial DNA (mtDNA) /C3a paracrine loop, restore the sensitivity of prostate cancer (PCa) cells to taxanes, and inhibit tumor expansion. Mechanistically, the mtDNA secreted by the PCa epithelium binds to the transmembrane protein DEC205 on the surface of CAFs, activating TLR9 and the maturation of the allergic toxin C3a, which enters TME and favors tumor cell proliferation and insensitivity to docetaxel [ 154 ]. A tumor immune barrier (TIB) formed by crosstalk between SPP1 macrophages and CAFs created an immunosuppressive microenvironment that hindered peripheral tumor infiltration of immune cells such as CD8 + T cells, thereby suppressing immunotherapy efficacy in hepatocellular carcinoma (HCC). Specifically, targeting SPP1 macrophages reduced the aggregation of CAF, again demonstrating the interaction between them [ 155 ]. Additionally, G-protein-coupled receptor 30 (GPR30) activated in CAF upregulated the expression and secretion of high mobility group protein 1 (HMGB1) in CAF. The overexpressed HMGB1 triggered the MEK/ERK signaling pathway and induced autophagy, which enhanced MCF-7 cell resistance to tamoxifen, thereby sparing BC cells from tamoxifen-mediated apoptosis [ 156 ]. As reviewed elsewhere, CAF can also bestow drug resistance upon tumor cells by regulating metabolism and inducing epigenetic modifications [ 157 , 158 ]. More recent findings suggested that ferroptosis may be involved in the treatment resistance as well. Ferroptosis was first proposed by Dr. Brent R.Stockwell in 2012 as a new manner of non-apoptosis, non-cellular necrosis, and iron-dependent cell death. The essence of ferroptosis is the inactivation of glutathione peroxidase, which leads to the accumulation of lipid peroxidation. Recently, researchers have discovered that CAF can inhibit ferroptosis in tumor cells through specific pathways. In GC, miR-522 secreted by CAF was a potential inhibitor of arachidonate lipoxygenase 15 (ALOX15), which was closely associated with toxic lipid peroxides. More importantly, this study demonstrated that paclitaxel and cisplatin could promote CAF secretion of miR-522 through the USP7/hnRNPA1 pathway, reducing chemotherapy sensitivity and revealing a new mechanism of chemotherapy resistance [ 159 ]. Similar results were found in glioblastoma (GBM). CAF upregulated the expression of lncRNA DLEU1 by activating HSF1, conferring ferroptosis resistance to GBM cells [ 160 ]. At last, it is also noteworthy that ECM deposition pertains to drug resistance. In PDAC, more than half (sometimes to 80% of the tumor mass) of the neoplastic tissues are composed of stromal tissues secreted by CAFs and other components. The hardened ECM can form a physical barrier, which hinders the arrival of chemotherapy and immunotherapy drugs to the cancer site via compressing peripheral blood vessels to reduce blood flow, thus attenuating the efficiency of drug delivery [ 6 , 161 , 162 ].
Immunosuppression
To survive and proliferate, CAFs must find ways to evade the immune system's surveillance at the cancer site. Although the intricate underlying mechanism of CAF suppressing immunity has not been fully understood, many studies have shown that CAF can suppress immunity in diverse ways. By secreting cytokines and chemokines like TGF-β and CXCL12, CAFs prevent the activation and recruitment of T lymphocytes in cancer sites [ 163 , 164 ]. Importantly, CXCL12 exerts its anti-inflammatory function in TME by inducing the transformation of T cells into Tregs, promoting the generation of macrophages that promote angiogenesis and dendritic cells (DCs) that are poorly functioning. Besides, the CXCL12/CXCR4 axis has been identified as associated with immune suppression and metastasis via recruiting immunosuppressive cells in numerous solid tumors. Recently, a study unraveled that a ketogenic diet (KD) has increased natural killer (NK) cell and cytolytic T lymphocyte (CTL) infiltration while improving immunosuppression by repressing CXCL12 in CRC. Mechanistically, KD significantly reduces the expression of KLF5 via increasing ketogenesis by overexpressing ketogenic enzyme 3-hydroxy-3-methylglutaryl-CoA synthase 2 (HMGCS2), which attenuates CXCL12 expression in CAF through binding to the CXCL12 promoter [ 164 , 165 , 166 , 167 ]. Of note, the TGF-β pathway can also directly promote the growth of CAFs, further influencing cancer progression. The overexpression of TGF-β can elicit CAF formation [ 168 ]. TGF-β1 can induce normal fibroblasts into CAFs in bladder cancer, and CAF proliferation has been significantly attenuated after using a TGF-β receptor inhibitor [ 169 , 170 ]. Besides, TGF-β1 affects EMT and invasion of BC cells through CAFs activation via overexpressing FAP and autophagy [ 171 ]. CAF-S1, a subset of myofibroblast, recruits CD4 + CD25 + T cells to create an immunosuppressive microenvironment via CXCL12 and expresses B7H3, CD73, and DPP4 to promote their differentiation into Tregs, thereby contributing to tumor growth [ 98 ]. Notably, the accumulation of myeloid-derived suppressor cells (MDSC) is considered a signal of increased immunosuppression. CAFs were reported to induce the differentiation of monocytes into MDSC via IL-6-mediated signal transducer and activator of transcription 3 (STAT3) activation manner [ 122 , 172 , 173 , 174 ], and FAP + CAFs can recruit MDSCs infiltration via STAT3-CCL2 signaling. Thus, it is persuasive that STAT3 hyperactivation can provide favorable conditions for CAFs to create an immunosuppressive microenvironment. More evidence indicated that in vitro and in vivo mouse BC models, CAF-intrinsic STAT3 activity exerts pro-tumorigenic functions through STAT3-dependent mediators like ANGPTL4, MMP13, and STC-1 [ 175 , 176 , 177 ].
The crosstalk between CAF and immune cells is gradually being unveiled. According to Kato T. et al . , CD8 + T lymphocytes and CAFs were negatively correlated in intratumoral tissues [ 178 ]. CAFs create an immune barrier to CD8 + T cell-mediated anti-tumor immune responses. It has been verified that CAF can diminish CD8 + T cell infiltration in tumors and contribute to ICB resistance [ 179 , 180 ]. CAFs even directly kill CD8 + T cells in an antigen-specific manner via PD-L2 and FASL [ 181 ]. In bladder cancer, FAP + CAFs were associated with poor infiltration of CD8 + T cells with stromal changes and significant loss of human leukocyte antigen (HLA-I) expression in cancer cells. Similar results were observed in HCC as well. Researchers showed that CAFs and M2 macrophages might pertain to CD8 + T cell exhaustion in steatotic HCC [ 182 ]. Another recent study found that apCAFs induced naive CD4 + T cells into Tregs, which disturbed the growth of CD8 + T cells in pancreatic cancer via IL-1 and TGFβ signaling pathways [ 183 ]. In stage-I lung squamous cell carcinoma (SqCC), PDPN + CAFs highly expressed TGF-β1 and recruit immunosuppressive cells like CD204 + tumor-associated macrophages [ 184 , 185 , 186 ]. Besides this, CAFs directly enhanced the recruitment of pro-tumoral immune cell populations, manifested by an increased Th2 response and a decreased Th1 response [ 187 ]. Th1 cells participated in the defense of the body from intracellular pathogens. By secreting TNF-α, TH1 cells inhibited the occurrence and development of tumors. Moreover, CAFs can highly express immune checkpoint ligands like PD-L1. PD-L1 suppresses anti-tumor immunity by binding to the receptor PD-1 on activated T lymphocytes to counteract T cell activation signals [ 187 , 188 ]. Similar results were reported by Dou D. and colleagues. CAF-derived exosome microRNA-92 increased the expression of PD-L1 in BC cells, which was correlated with impaired T cell proliferation. Animal studies conducted by the same group further confirmed the functional impairment of tumor-infiltrated immune cells in vivo [ 189 ]. In addition, four CAF subtype populations were identified in NSCLC by paired scRNA-seq and IHC analysis. In tumor lesions containing MYH11 + αSMA + CAF and FAP + αSMA + CAF, the density of CD3 + or CD8 + T cells was remarkably reduced compared to T cell-permissive CAFs, indicating that both CAFs were associated with T cell exclusion [ 190 ].
Overall, existing studies have explored the pro-tumor function of CAF in multiple ways. In practice, the detailed mechanisms responsible for the biological pro-tumor role of CAFs still need to be discussed meticulously. Nevertheless, the contribution of CAFs to tumor progression mentioned above is just the tip of the iceberg, multiple signaling pathways were demonstrated to pertain to the pro-tumor functions of CAFs (Fig. 3 ). New research from Sazeides, C. & Le, A. suggests that exosomes derived from CAFs (CDEs) contribute to reprograming cancer cells' metabolic activity via downregulating specific genes [ 184 , 191 ]. Moreover, cancer stem cells (CSCs) were found to be regulated by TME components like CAF. CAFs maintain transfer colonization of CSCs via periostin. BCSCs also express the Hh ligand Shh, which enables CAF’s expansion through paracrine. In PC, researchers revealed that CAFs could facilitate cancer stemness via the OPN / SPP1-CD44 axis, and the promoting effects were counteracted after a specific blockade. Even so, the interactions between CAFs and CSCs have yet to be discovered [ 192 , 193 ]. Still, most of the experiments to validate the cancer-promoting functions of CAF were done in xenotransplantation or co-implantation models, which might cause errors or deviations in the transcriptional process of CAF biomarkers. Consequently, the fundamental mechanisms of CAFs in human tumors remain to be confirmed.

CAF interacts with a variety of tumor-promoting components through multiple signaling pathways. CAF can exert its pro-tumor function by promoting tumor neovascularization, promoting tumor cell proliferation and metastasis, regulating tumor microenvironment to an immunosuppressive state, and reconstructing ECM, etc. CAF, cancer-associated fibroblast; CTGF, connective tissue growth factor; CXCL, C–X–C motif chemokine; EGFR, epidermal growth factor receptor; FGF, fibroblast growth factor; HGF, hepatocyte growth factor; HMGB1, high mobility group protein 1; IGF, insulin-like growth factor; IL, interleukin; NOTCH3, neurogenic locus notch homolog protein 3; LOXL2, lysyl oxidase like 2; MMP, matrix metalloproteinase; PDGF, platelet-derived growth factor; PGE2, prostaglandin E2; POSTN, periostin; SDF-1, super dimensional fortress-1; SPARC, secreted protein acidic and cysteine rich; TGF-β, transforming growth factor beta; TNFSF4, tumor necrosis factor superfamily member 4; VEGF, vascular endothelial growth factor. By Figdraw
The cancer-restraining functions of CAFs
In line with the functional heterogeneity of CAF mentioned earlier, notwithstanding the majority of the existing studies have focused on the various pro-tumor functions of CAFs, the inhibitory functions of CAF on cancer should not be neglected and remain to be elucidated in more detail. Recent experiments have detected the expression of CD9, CD63, and CD81 in CAF-derived exosomes by western blotting. Surprisingly, CD9-positive exosomes can inhibit the proliferation of malignant melanoma. This study also highlighted that patients with CD9-positive exosomes showed longer five-year survival rates [ 194 ]. Evidence from recent studies has also demonstrated that CAF can remarkably improve drug sensitivity. In lung cancer, a particular subset of CAF: CD200-positive CAF was uncovered to elevate the sensitivity of cancer cells to EGFR-tyrosine kinase inhibitor (EGFR-TKI), gefitinib, and the sensitizing potential was deprived when CD200 was knocked out [ 195 ]. Another study unraveled that when CAF was co-cultured with NSCLC cells, the secretion of IGF and IGF-binding proteins (IGFBPs) was linked to the drug sensitization of EGFR-TKI [ 196 ]. As mentioned, deleting αSMA + myCAFs in PDAC increases immunosuppression and reduces OS. A previous study has reported that CAFs can impede PDAC progression by hypoxia. Meanwhile, according to Rhim, A. D. et al . , Sonic hedgehog (Shh) is a soluble ligand overexpressed in PDAC tumor cells that promotes the formation of the fibroblast-rich stroma. Deleting SHH in murine models did reduce the interstitium of tumors, but at the same time, SHH-deletion tumors also showed more extraordinary proliferative ability and aggressiveness. In a way, myCAFs exert tumor suppressor function partially through the SHH-SMO signaling pathway [ 5 , 7 , 12 , 197 , 198 ]. Simultaneously, Bhattacharjee and colleagues have demonstrated that myCAF-expressed type I collagen can physically restrict desmoplastic tumor growth. They found that deletion of type I collagen in mice models tremendously promoted tumor metastasis in PDAC and CRC. A possible mechanism is that type I collagen establishes a mechanical barrier that limits tumor growth [ 13 ]. Again, Tanaka, R. and colleagues recently revealed that CAF-secreted IL-8 had a suppressive effect on the proliferation of OCUCh-LM1 cell lines associated with tumor formation [ 199 , 200 ]. Since apCAFs have been reported to present antigens to CD4 + T cells and are therefore believed to be involved in the anti-tumor process, more evidence is anticipated to fully unravel its anti-tumor role [ 92 ]. Cumulatively, the above evidence indicates that CAF also has a potential anti-tumor function that should not be overlooked.
Advances in targeted CAF strategies in different cancers
Collectively, the multifaceted tumor-promoting functions that CAFs exhibit during tumor progression make them appealing therapeutic targets for oncotherapy. The easiest way to target CAF is to eradicate CAF or make it functionally impaired. Endo180 is a circulating endocytosis receptor expressed exclusively in fibroblasts, with higher expression in CAF populations than in normal fibroblasts. Studies have shown that tumor growth and progression were immensely limited in Endo180 −/− mice due to genetic deletion of the Endo180 receptor. This pro-tumor functional damage was caused by CAF intrinsic contractility defects and decreased CAF activity [ 180 , 201 , 202 ]. One major approach to eliminate CAFs is to target specific surface markers. For instance, FAP is expressed on a subset of CAFs in different tumors. Chimeric antigen receptor T cell treatment (CAR-T) can explicitly target CAFs. From previously published studies, FAP-specific CAR-T cells can kill most FAP + cells, including CAFs, and prevent the growth of tumor stroma, which enhances the absorption of chemotherapy drugs and has anti-tumor benefits. FAP-expressing cells in the tumor microenvironment have been specifically and directly removed using infrared photoimmunotherapy (NIR-PIT), a new and novel method to remove CAFs. This approach inhibited tumor growth in a co-cultured human esophageal squamous cell carcinoma xenograft model without adverse effects. So far, the FDA (Food and Drug Administration) has approved five CAR-T therapies for hematological malignancies of B cell origin. In contrast, no CAR therapy has been approved for solid tumors yet [ 203 ]. Nevertheless, obtaining clinical benefits is not necessarily limited to completely eradicating or reprogramming CAF but can be achieved by blocking signals from CAF. In that signaling pathways are partially related to CAF, some promote the growth, proliferation, invasion, and metastasis of CAF through the secretion of various factors. Other pathways expressed by CAF modulate or transform the TME to make it generally conducive to tumor growth. Moreover, numerous anticancer medications undergoing human testing may also target CAF or its metabolites. Histone deacetylase (HDAC) and SMO inhibitors have undergone extensive testing in numerous clinical trials. These medications alter intracellular signaling and epigenetic regulation in tumor cells, CAFs, and CAF precursors [ 204 ]. It is important to note that CAF.ERα( +) (estrogen receptor alpha) can impede the metastasis and invasion of prostate cancer by inhibiting macrophage infiltration and modulating the expression of thrombospondin 2 (Thbs2) and MMPs [ 205 , 206 ], which emphasizes the need for caution in targeting CAF. As our understanding of CAF biology in cancer deepens, CAF-targeted therapies are gradually being reinvigorated, and many clinical trials are underway. Next, we will comprehensively introduce advances in targeting CAFs in several types of cancer.
Breast cancer
Breast cancer, a malignant tumor that seriously endangers women's health and is occasionally seen in males, has become a public health issue worldwide [ 207 , 208 , 209 ]. In 2020, it was the most diagnosed malignancy [ 210 ]. According to the difference in the expression level of different hormone receptors: ERα (estrogen receptor α), PR (progesterone receptor), and HER2 (human epidermal growth factor receptor 2), BC can be briefly classified into four types: luminal A, luminal B, HER2‐positive, and triple‐negative, and of course, the prognosis of each varies [ 122 , 211 ]. Today's main treatments for breast cancer are radiotherapy, chemotherapy, endocrine therapy, surgery, or a combination of these. Despite all the progress made in the past decade, the incidence rate of BC has risen continuously. Targeting CAF therapy may shed light on the current clinical BC treatment.
Targeting CAFs specific molecules and biomarkers
As a significant biomarker of CAFs and an emerging cancer promotor, FAP is deemed one of the most feasible and clinically useful CAF markers. Thus, innumerable studies have been designed to look into FAP in recent years. Administration of an anti-FAP monoclonal antibody (mAb), FAP5-DM, has provided long-lasting inhibition of tumor growth and even complete tumor regression with no signs of intolerability in stroma-rich xenograft models of various cancers [ 212 ]. In another mouse 4T1 metastatic BC model, researchers developed a FAP-targeting immunotoxin αFAP-PE38 to deplete FAP-positive stromal cells, which showed efficacy in suppressing tumor growth [ 213 ]. Of note, FAP-targeted vaccines have shown their antitumor function in both in vitro and in vivo experiments, modulating the immunosuppressive microenvironment and decreasing tumor growth and angiogenesis [ 122 , 214 ]. To date, several FAP-based vaccines have been investigated in preclinical trials. Administration of oral FAP DNA vaccine induced CD8 + T cell–mediated killing of CAFs and successfully suppressed primarytumor growth and colon and breast carcinoma metastasis in multidrug-resistant murine models. DNA vaccine can remarkably decrease stroma type I collagen expression and improve the efficacy of chemotherapy [ 215 ]. Recently, a synthetic consensus (SynCon) FAP DNA vaccine has displayed superiority at breaking immune tolerance compared to the native FAP immunogen in genetically diverse mice. The SynCon FAP DNA vaccine synergized with other tumor-antigen-specific DNA vaccines showed a stronger anti-tumor activity than monotherapy, and the SynCon FAP DNA vaccine itself exerted remarkable antitumor effect in the TC-1, Brpkp110, and TSA tumor models [ 216 ]. So far, the use of DNA vaccines has been limited to animal experiments, and no DNA vaccines have moved into the clinic. Researchers have developed an FAP.291-based epitope minigene vaccine that can activate CTL against CAFs and suppress tumor progression in murine BC models [ 217 ]. Moreover, several drugs targeting FAP have been submitted to clinical trial-enrolled patients with metastatic CRC, including Sibrotuzumab (a FAP targeting humanized monoclonal antibody) and Talabostat [ 218 , 219 ]. However, all these drugs failed to pass clinical phase II trials. As reviewed elsewhere, targeting FAP molecular imaging is also booming in diagnostic imaging. For instance, in PET (positron emission tomography)/CT (computed tomography), 68 Ga-FAPI-04, one of the quinoline-based FAP inhibitors (FAPIs) developed by the University Hospital Heidelberg, has become a more promising tracer that can discriminate cancerous lesions more accurately compared with 18 F-FDG in a cohort of 48 BC patients. At the same time, the FAPI series has certain limitations in tumor retention. Consequently, a compound FAP-2286 was developed to overcome the obstacle, and 68 Ga-FAP-2286 has demonstrated its ability for imaging in preclinical models, not just in BC [ 57 , 220 , 221 , 222 ]. Except for being tumor-promoting, FAP was also observed to have some tumor-inhibiting properties. A second independent observation found that more abundant FAP of invasive breast ductal carcinoma is associated with longer overall and disease-free survival [ 223 ]. These studies confirmed the functional heterogeneity of CAFs, which was probably related to the failure of clinical trials mentioned before. It is noteworthy that CAFs do not exclusively express FAP, so the shortage of CAF-specific biomarkers greatly hindered the precision targeting of CAFs via the abovementioned approaches. Therefore, other FAP-expressing cells may also be influenced when using FAP-specific strategies to delete CAFs, leading to adverse consequences. For instance, due to the killing of multipotent bone marrow cells that express low levels of FAP, FAP CAR-T cells induced significant cachexia and lethal bone toxicities in mouse strains bearing a variety of subcutaneous tumors [ 224 ]. Thus, finding biomarkers exclusively expressed in CAFs is imperative for CAF-targeted oncotherapy. Alternatively, about 20% ~ 30% of BC patients' tumors are HER-2 positive type. The HER2-positive subtype, characterized by ERBB2 amplification, has a poorer clinical prognosis than HER2-negative tumors and is prone to recurrence [ 225 , 226 ]. Anti-HER2 mAbs like trastuzumab and pertuzumab are one of the main therapeutic agents in first-line therapy. However, half of the HER2-positive patients benefit little to no from HER2-targeted therapy, and one in five patients will relapse after treatment. Studies have highlighted that CAFs play an essential role in the anti-HER2-targeted therapies resistance. Rivas, E. I. et al . revealed that aggregation of CAFS 1 and pCAF in the CAF subtype of BC was significantly increased in patients who did not respond to anti-HER 2 mAb therapy, potentially leading to reduced IL2 activity. In contrast, low IL2 activity may be associated with treatment resistance. Besides, FAP is the biomarker expressed by both CAF S1 and pCAF, they found that IL2 activity was maintained using a novel immunocytokine FAP-IL2v, Simlukafusp Alfa. In vitro models, this monoclonal antibody fusion protein consisting of an IL-2 variant and a FAP-targeting protein has been shown to enhance antibody-dependent cellular cytotoxicity (ADCC) by activating NK cells. It is currently undergoing evaluation in a phase I clinical trial in combination with trastuzumab. In addition, in murine models of multiple human cancers, FAP-IL2v combined with various therapeutic antibodies has also shown some positive efficacies [ 226 , 227 , 228 ].
Targeting CAF-associated signaling pathways
Targeting CAF-associated signaling pathway therapy should not be dismissed, the TGF-β signaling pathway has attracted the attention of oncologists in the past decade. The links between TGF-β and CAF include (1) CAF paracrine TGF-β can induce EMT of breast cells, promote the transformation of BC cell lines to a more invasive phenotype, and activate the TGF-β/Smad pathway. CAFs are reported to activate the transcription of HOTAIR through TGF-β1 secretion to promote BC cell metastasis; (2) the autocrine TGF-β1/miR-200 s/miR-221/DNMT3B loop maintains CAF activity and promotes BC progression, and destroying the loop can restore the NF phenotype; (3) TGFBR2 expressed by CAF affects the growth and survival of BC cells; (4) the elevated level of TGF-β transcription in BC stimulates the conversion of NFs to CAFs, and gene ZNF32 prevents NF-to-CAF conversion by directly binding to the TGFB1 promoter to inhibit the transcription process; (5) the hyperactivity of TGF-β signaling pathway in CAF is often associated with immunotherapy failure [ 229 , 230 , 231 , 232 , 233 , 234 ]. Therefore, a number of TGF-β pathway inhibitors were developed. Fresolimumab, a neutralizing antibody that targets TGF-β1,2,3, has confirmed its anti-tumor feasibility and safety in a phase II clinical trial (NCT01401062), in which researchers focused on the cooperation of Fresolimumab and focal irradiation while applying to 23 patients with metastatic BC. Participants were divided into two groups, receiving different doses of Fresolimumab. Seven grade 3/4 adverse events occurred in 5 of 11 patients in the 1 mg/kg group and 2 of 12 patients in the 10 mg/kg group, respectively. Higher doses of Fresolimumab were shown to improve median OS, as the median OS was reported to be 7.57 months in the low-dose group compared to 16.00 months in the high-dose group. [ 229 , 235 , 236 ]. The combination of TGF-β receptor I kinase inhibitor Galunisertib (LY2157299) and PD-L1 blockade has also shown excellent results in tumor treatment. To date, Bintrafusp alfa (BA), a fusion protein that can simultaneously inhibit both TGF-β and PD-L1 pathway, was demonstrated to possess a stronger affinity with TGF-β1 and inhibition of cancer cell proliferation than Fresolimumab in MC38 tumors [ 237 , 238 ]. In addition, targeting TGF- β1 was regarded as a method to solve chemoresistance in CAFs [ 239 ]. It is intriguing that losartan, the first angiotensin II receptor antagonist, typically known as the antihypertensive drug, can downregulate the TGF-β pathway and inactivate CAFs. Patients with triple-negative breast cancer (TNBC) are relatively resistant to anti-PD1 therapy. Zhao Q and colleagues proposed a combined therapy of Losartan, doxorubicin hydrochloride liposome (Dox-L), and α-PD1, which results in reduced ECM and better regulation of the immune microenvironment, may guide the clinical treatment regimen of TNBC. Moreover, losartan has been reported to improve delivery efficiency and the therapeutic effect of photodynamic nanoplatforms by depleting tumor collagen [ 240 , 241 , 242 ]. Natural compounds like Zerumbone (ZER) were found to repress BC cell metastasis via downregulating mRNA transcription. ZER has been shown to reduce the neoplasticity and motility of TNBC cells by inhibiting the TGF-β1 signaling pathway and can increase the sensitivity of BC cells to paclitaxel [ 243 , 244 , 245 , 246 ].
CXCR4 has been described to promote BC cell proliferation and expedite tumor growth via recruiting immune cells and facilitating angiogenesis [ 247 ]. The CXCL12/CXCR4 pathway has emerged as a vital part of BC tumorigenesis and in BC metastasis to the brain, liver, and lung in the past few years [ 248 , 249 , 250 ]. It is reported that the recruitment of endothelial progenitor cells (EPCs) was mediated by CAF-derived CXCL12, which promoted angiogenesis in BC, and CXCL12 secreted by CAF also directly stimulated tumor growth. Autocrine CXCL12 signaling in breast fibroblasts initiated and maintained the pro-tumor CAF phenotype [ 230 , 251 ]. In addition, due to the CXCL12-CXCR4 axis driven by CAF, monocytes were recruited into tumor sites to acquire the tumor-promoting ability of lipid-associated macrophage to maintain the immune microenvironment in a suppressed state [ 252 ]. The CXCR4 antagonist AMD3100 was observed to increase CTL infiltration and reduce desmoplasia and immunosuppression in mouse metastatic BC models [ 253 ]. AMD3100 also attenuated TNBC cell migration and metastasis in zebrafish embryos [ 254 ]. Similarly, Combination therapies have shown promising results. In an animal experiment, AMD3100 and tamoxifen significantly alleviated tamoxifen resistance without obvious side effects [ 255 ]. The combination of AMD3100 and PARP1 inhibitor, Olaparib was found to have a positive correlation and can suppress tumor growth and metastasis in vivo TNBC animal experiments via inducing severe DNA damage [ 256 ]. AMD3100 combined with anti-PD-1 therapy has proven useful more than just in murine BC models [ 124 , 257 , 258 , 259 ]. Wu, Y. et al . elucidated that the blockade of FGFR signaling by Erdafitinib mechanically degraded the secretion of vascular cell adhesion molecule 1 (VCAM-1) through downregulating MAPK/ERK pathway in CAFs, creating a favorable microenvironment for T cell infiltration [ 260 ]. Furthermore, CAFs were found to have close communication with BC cells via the HGF-MET pathway. Blocking HGF-MET signaling can simultaneously target primary TNBC tumorigenesis and lung metastasis in a three-dimensional organotypic tumor model and alleviate radioresistance [ 261 , 262 ]. Analogically, the potential of the HGF-MET pathway as a therapeutic target was discovered in NSCLC and prostate cancer [ 263 , 264 ].
As for the STAT3 signaling pathway, it has been shown that CAF-secreted TIMP-1 activated the STAT3 pathway in BC cells, promoting proliferation and migration, and CAF-derived IL-6 can increase extracellular TIMP-1 abundance, suggesting that inhibition of TIMP-1/CD63/integrin β1/STAT3 loop may be a promising therapeutic modality. In addition, CAF-derived IL-6 can directly activate the STAT3 pathway, promoting the growth and radioresistance of BC cells [ 265 , 266 ]. The primary representative inhibitors can be divided into peptides, small molecules, and oligonucleotides. In pre-clinical cancer models, Peptides such as ISS-610 prodrugs and small molecules like compound 6o, Stattic, and FLLL32 were demonstrated to generally upregulate apoptosis of BC cells [ 267 , 268 , 269 ]. Similarly, Stattic can resensitize BC cells to tamoxifen by inhibiting cell proliferation and inducing apoptosis in tamoxifen-resistant cell lines [ 270 ]. Of note, Tocilizumab (TCZ) continuously inhibited CAF biomarkers beyond STAT3 in situ, humanized breast tumors in mice, but also reduced tumor angiogenesis and metastasis [ 271 ]. A phase 1 study (NCT03135171) enrolled 11 patients with BC to determine the safety and tolerability of the cooperation of tocilizumab, trastuzumab, and pertuzumab has recently been completed, and the result is about to be available. Alternatively, STAT3 inhibition through siRNA suppressed cancer cell proliferation and resensitized neuroendocrine tumors to mTOR inhibitor Everolimus treatment [ 272 ]. Of note, Hu, G. et al . unravel that CD73 + γδTregs was the dominant regulatory T cell in human BC and was associated with poor clinical outcomes. Mechanistically, the IL6-adenosine positive feedback loop formed between CD73 + γδTregs and CAFs promoted the production of the immunosuppressive microenvironment and accelerated tumor progression [ 273 ]. At last, the focal adhesion kinase (FAK) signaling pathway was known for its profibrotic function and could become a drug target. Zhang and colleagues suggested that zeste homolog 2 (EZH2) activating TGF-β signaling via activating FAK signaling, using FAK inhibitors, can effectively inhibit BC bone metastasis in vivo [ 226 , 241 , 274 ].
Targeting stroma
Another target should be stroma proteins. The desmoplasia response is due to the deposition of large amounts of ECM proteins, such as fibro collagen, hyaluronic acid, and tenascin C, as well as CAF-mediated ECM reshaping. Therefore, some strategies to improve ECM stiffness, including targeting the production of ECM proteins or degrading ECM, are seen as effective protocols for targeting CAF [ 49 , 275 ]. Tenascin-C (TNC) is a hexamer, multi-module extracellular matrix protein. It comes in various molecular forms and is produced by alternative splicing and protein modification [ 276 ]. TNC has been identified to regulate tumor angiogenesis and tumor immunity, especially the function of CTL, plasticity, and tumor metastasis in multiple cancers [ 276 , 277 , 278 , 279 , 280 ]. According to Murdamoothoo, D. et al . , TNC can retain CD8 + TIL in cancer stroma by binding CXCL12, which facilitates the progression of BC. By blocking CXCR4 with AMD3100 in murine models, CD8 + TIL and macrophage infiltration are promoted, causing tumor cell death [ 281 ]. Besides, in TNBC, researchers have revealed that high Tenascin-C expression correlated with poor prognosis in TNBC patients using Kaplan–Meier meta-analyses and was negatively associated with LC 3B expression and CD8 + T cells. Targeting TNC enables TNBC cells sensitive to checkpoint inhibitors and sensitizes PD-1 blockade therapy in mice models [ 282 ]. Several clinical trials and animal experiments on inhibiting the TNC pathway or targeting TNC and other combined factors have begun to bear fruit [ 283 , 284 ].
Other targets of CAFs
It is also noteworthy that ceramide accumulation can upregulate the expression of tumor suppressor gene p53 and restrain CAF activation during sphingosine kinase 2 (SphK2) deletion. The utilization of SphK2 inhibitors caused ECM reprogramming, manifested by increased expression of matrix P53, restriction of fibroblast conversion to CAF, and ultimately impaired cancer progression [ 285 ]. Breast CAFs typically express the MCL-1 gene, and the MCL-1 expression level in breast CAFs is higher than in normal fibroblasts. In luminal breast cancers, MCL-1 expression is influenced by paracrine effects. Bonneaud and colleagues unraveled that targeting MCL-1 via BH3 mimetic, an antagonist to various BCL-2 congeners, including MCL-1, can mitigate the invasion of cancer cells and inhibit their tumor-promoting function. A possible mechanism is that BH3 mimetic promotes mitochondrial cleavage in bCAF [ 286 ]. Exosome-based research is in full swing, and exosome-based nucleic acid delivery has become an emerging cancer treatment option [ 287 ]. It has been suggested that inhibition of CAF-derived exosomes like miR 500a-5p and miR-92 can suppress the growth and metastasis of BC and may be a potential therapeutic target [ 189 , 288 ]. Conversely, the loss of miR-4516 leads to malignancy in TNBC, suggesting that miR-4516 can potentially become an antitumor drug for TNBC [ 289 ]. In addition to drug targets, researchers have also found that CAFs and specific markers can indicate and predict prognosis. The AU-rich RNA-binding factor 1 (AUF1), Podoplanin, and ATR-negative CAFs have been shown to correlate with poor OS [ 290 , 291 , 292 ].
Pancreatic cancer
PDAC, characterized by fibroinflammatory hyperplasia, has the potential for early metastasis and accounts for more than 90% of all pancreatic malignancies. However, magnificent resistance to existing treatments such as chemotherapy, radiotherapy, and molecularly targeted therapy makes pharmacological treatment of PDAC formidable and prone to relapse. All these factors contribute to a poor prognosis for PDAC, with a five-year survival rate of less than 10% [ 293 ]. The progression of PDAC is intimately intertwined with CAFs and immunosuppressive cells such as Tregs and TAMs. Also, crosstalk between CAF and tumor cells complicates the PDAC tumor microenvironment and favors drug resistance. Therefore, novel strategies targeting CAFs have piqued researchers’ interest.
Targeting CAF-related proteins
The leucine-rich repeat-containing protein 15 (LRRC15), a marker of mesenchymal stem cells (MSCs) that belonged to the LRR family, was highly and exclusively expressed in CAFs under TGFβ regulation in lots of mesenchymal-derived tumors and solid tumors. LRRC15 + CAF have been found to hamper the function of CD8 + T cells, and specific consumption of LRRC15 + CAF using Lrrc15-diphtheria toxin receptor inhibited tumor growth and increased ICB response [ 294 ]. Furthermore, the anti-LRRC15 antibody ABBV-085 has shown efficacious in tumor regression, and then the combination therapy expanded the therapeutic benefits [ 295 , 296 ]. To date, ABBV-085 has been investigated in a phase I clinical trial (NCT02565758) which enrolled 85 patients with advanced solid tumors to evaluate the safety and pharmacokinetics of ABBV-085 and determine the dose. However, no results have been posted. More importantly, a recent scRNA-seq analysis of fibroblasts from normal pancreas and PDAC provided insights into fibroblast evolution during tumor progression, identifying LRRC15 + CAFs of prognostic significance in immunotherapy clinical trials [ 97 ]. SLC7A11 (xCT) is a cystine transporter whose therapeutic potential has been established in PDAC. A study found that SLC7A11 abrogation tremendously decreased tumor growth and CAF activation in vitro and in vivo, making targeting SLC7A11 treatment in PDAC-derived CAF a potential therapy [ 297 , 298 ]. Another glutamatergic pre-synaptic protein Netrin G1(NetG1), was also overexpressed in CAF, associated with CAF metabolism and immunosuppression. Inhibition of this fibroblastic target with neutralizing monoclonal antibody in vivo has been shown to reverse the tumor-promoting function of CAF and change its immunosuppressive function in preclinical mouse models [ 299 ]. As mentioned earlier, HSPG2 was expressed by mutant Tp53 to educate CAFs (mt-e-CAF) and contribute significantly to tumor metastasis. The researchers showed that consuming perlecan in combination with chemotherapy prolonged the survival of mice. Given that perlecan expression is predominantly mediated by nuclear factor kappa-B (NFκB) signaling, NFκB inhibitors were proposed as a possible agent to decrease perlecan in pancreatic CAF, and the therapeutic value of perlecan was also discovered in TNBC. Moreover, anti-HSPG drugs such as necuparanib have been reported to lead to MMP1 activity restriction and tissue inhibitor of metalloproteinase 3 (TIMP3) increase in PC patients [ 129 , 130 , 300 , 301 , 302 ]. Nevertheless, PDAC is primarily characterized by fibrous hyperplasia, closely related to PSC and CAFs. Heat shock protein 90 (HSP90) has been shown to play an essential role in tumor and immune system regulation. Despite the crosstalk between PSC/CAF and HSP90 being still unclear, HSP90 inhibition by XL888 can attenuate tumor growth in vitro and enhance the efficacy of anti-PD1 therapy in vivo, which may guide the subsequent direction of research [ 303 , 304 ].
Reprogramming CAF and targeting CAF-associated signaling pathways
In addition to depleting CAFs via their biomarkers or related proteins, another strategy to target CAFs is to weaken or eliminate their pro-tumorigenic functions. In this scenario, some studies have reprogrammed activated CAFs to quiescence, even converting their tumor-promoting phenotypes to tumor-suppressing phenotypes. Since vitamin A deficiency in patients with PDAC leads to PSC activation, restoring retinol stores in PSC by ATRA may reset PSC into a quiescent phenotype. Such reversion of PSCs facilitated apoptosis of surrounding PC cells and decreased proliferation via inhibiting Wnt-β-Catenin Signaling [ 305 ]. The combination of ATRA and gemcitabine was found effective in restraining PDAC progression in mouse models. This combination was mediated through a range of signaling cascades (Wnt, hedgehog, retinoid, and FGF) in cancer and stellate cells [ 306 ]. Moreover, a phase Ib clinical trial for patients with advanced, unresectable PDAC demonstrated that re-purposing ATRA as a stromal-targeting agent with gemcitabine-nab-paclitaxel is safe and tolerable [ 307 ]. These outcomes offer enormous opportunities for ATRA to act as potential drugs to treat PC. Besides, in another study, pharmacologically stimulating vitamin D receptors (VDR, a master genomic suppressor of activated PSCs) by VDR ligand calcipotriol successfully inactivated PSCs. VDR ligand-induced stromal reprogramming reduced cancer-associated fibrosis and inflammation and enhanced the efficacy of a co-administered chemotoxic agent, gemcitabine [ 308 ]. Of note, pharmacological reprogramming of CAFs has only been achieved in PDAC contexts. More research is needed to determine whether the reprogramming strategy works in other cancer types.
Similar to previous descriptions of BC, drugs targeting the CXCL12-CXCR4 axis also played a non-negligible role in PC treatment. As AMD3100 synergistic anti-PD1 therapy has been shown to attenuate the number of cancer cells in mouse models significantly, a phase 2 trial (NCT04177810) was conducted in patients with metastatic PC to investigate the combination efficacy of AMD3100 and cemiplimab. The study enrolled 25 participants who will be administered Cemiplimab intravenously (350 mg) on day 1 in a 21-day cycle and AMD3100 at a dose of 80mcg/kg/hr as a continuous intravenous infusion of the first 7 days of each cycle, no results posted [ 309 ]. Importantly, AMD3100 was able to deal with immune suppression. Continuous infusion of AMD3100 induced intratumoral T lymphocyte aggregation in patients. It unexpectedly activated the B-cell response, and most patients showed an enhanced T, B cell response after only one week [ 257 , 310 ]. Based on the immunosuppressive microenvironment of PC, some patients have little or no response to immunotherapy. Of note, a mutual feature of decreased expression of the chemokine CXCL12 was observed in a subpopulation of resistant cells in KPC mice models. Additionally, the use of AMD3100 changed the phenomenon that adding CXCL12 reversed the resistant phenotype, indicating that lack of CXCR12 might cause drug resistance in human PC. However, a phase 1/2 study (NCT02472977) of the safety and therapeutic efficacy of anti-PD-1 antibody nivolumab and anti-CXCR4 monoclonal antibody ulocuplumab was terminated due to lack of efficacy in the short-term acute phase [ 311 ]. These pieces of evidence suggested altogether that the sophisticated mechanisms of the CXCL12-CXCR4 axis are still not fully learned and require more in-depth study and dissection. Notable, polymeric AMD3100 (PAMD) was reported to enhance drug delivery of siRNA nanoparticles in PDAC. For instance, PAMD modified with hydrophobic tetrafluoro-p-toluic acid (TFTA) and conjugation of α-tocopherol (TOC) to PAMD indicated higher cellular uptake and tumor accumulation [ 312 , 313 ]. Another phase 2 clinical trial (NCT02826486) enrolled 80 participants with metastatic pancreatic adenocarcinoma has been designed to investigate the combination of CXCR4 inhibitor BL-8040 (motixafortide), anti-PD1 mAb pembrolizumab, and chemotherapy of onivyde. Although the outcome remains unknown, it is expected to increase patients’ objective response rate (ORR). In addition, a phase II clinical trial of triple therapy, including mopisafortide, pembrolizumab, and NAPOLI-1 regimens (nanoliposomal irinotecan, fluorouracil, and calcium folinate), is safe, effective, and tolerable [ 314 , 315 ]. A phase II study (NCT04543071) with BL-8040, cemiplimab, and combination chemotherapy (Gemcitabine and Nab-Paclitaxel) in pancreas adenocarcinoma is now recruiting. What’s more? Unlike AMD3100, which was found to affect heart rhythm and cause hypotension in a clinical trial (NCT01280955), MSX-122, another small non-peptide molecule, exhibited fewer side effects than AMD3100. Still, the reason why the MSX-122 clinical trial was discontinued remains unknown (NCT00591682) [ 316 ]. Based on the current findings, STAT3 signaling pertains to PDAC drug resistance. CAF-derived circFARP1 synergistically increases the expression and secretion of leukemia inhibitory factor (LIF) by inhibiting CAV1 degradation and acting as a miR-660-3p sponge, activating the STAT3 pathway in PDAC cells, leading to gemcitabine resistance [ 317 ]. Moreover, dual inhibition of MEK and STAT3 pathway with specific inhibitors showed reduced stromal inflammation and enrichment of mesenchymal stem cell-like CAF phenotypes, alleviating PDAC immunotherapy resistance.
The hedgehog (Hh) signaling pathway is instrumental in embryonic development and tissue patterning. Usually, the Hh signaling pathway in adults is almost entirely silent in tissues, and abnormal activation of the Hh signaling pathway can lead to carcinogenesis. Constitutive activation of the Hh signaling pathway is explicitly associated with cancer development and progression of various solid malignancies, such as basal cell carcinoma (BCC), medulloblastoma (MB), PC, PCa, etc. [ 318 , 319 ]. SHH pathway was demonstrated to be directly or indirectly involved in tumor angiogenesis, and activation of Hh signaling in CAF can upregulate the expression of CXCR4 and IGF1R in TME. Again, it also triggered CAF Gli1 upregulation and impacted the expression of transcription factor snails in PC cells through paracrine action, enhancing EMT in PC cells [ 320 , 321 , 322 ]. Therefore, inhibition of the Hh signaling pathway has emerged as an attractive and promising cancer therapeutic strategy. The Hh signaling pathway can be divided into two different pathways: canonical and noncanonical. In the canonical pathway, cancer-derived sonic hedgehog (SHH) is an activating ligand for transmembrane protein smoothened (SMO), which is present on neighboring CAFs and promotes ECM production [ 12 ]. As an essential molecule, SMO is involved in cascade and has become the primary target of Hh signaling pathway inhibitors [ 323 ]. So far, few SMO inhibitor has been approved by FDA for oncotherapy. Vismodegib (GDC-0449) is the first FDA-approved SMO inhibitor for treating advanced and metastatic BCC. Sonidegib (LED-225), another potent SMO inhibitor, received FDA approval in 2015 as a new treatment for locally advanced or metastatic basal cell carcinoma. Although CAF-associated SHH signaling is one of the main pathways of the stromal proliferation of PDAC, clinical trials on SHH pathway inhibitors are not progressing well. In 2014, the deletion of SHH in mouse models and the pharmacological reduction of SHH pathways using vismodegib was pointed out by scientists not only did not have the desired tumor suppressive effect but, in some contexts, accelerated the tumor process because it reduced the stromal desmoplasia [ 12 , 197 ]. The SMO inhibitor Sonidegib has been reported to alter the proportion of CAF subsets by increasing the number of iCAFs and decreasing the number of myCAFs, thereby attenuating CTL aggregation, which is aligned with increased immunosuppression and may lead to worse long-term consequences [ 324 ]. Currently, major drugs targeting the SHH pathway can be classified into SMO inhibitors, HHAT inhibitors, anti-HH mAbs, and GLI inhibitors. However, few of them were demonstrated to alleviate PDAC [ 325 , 326 , 327 ]. Despite all these frustrating facts, studies still prove the anti-tumor effects of SHH inhibitors in PDAC. RU-SKI 43, an HHAT inhibitor, was reported to hinder PC cell growth via smoothing-independent non-canonical signaling and could potentially treat leiomyosarcoma, according to Ph, S., and colleagues [ 328 , 329 ]. Moreover, combination therapies have exhibited some promising results. In PC mouse models, simultaneous targeting of CXCR4 and hedgehog pathways with AMD3100 and vismodegib can improve the therapeutic efficacy of gemcitabine. A more significant reduction in Ki67-positive cells was observed in triple-treatment mice compared with gemcitabine alone, indicating that tumor growth was almost completely inhibited, which solved the problem of chemotherapy resistance to some extent [ 330 ]. Another combination of PEG-Gem-cisPt-MSNs and synthetic consisting of SHH inhibitor, cyclopamine (CyP), and mesoporous silica nanoparticles (MSN) have been corroborated in vivo evaluation to enhance the efficiency of drug delivery to tumor cells and reduce tumor mass [ 331 ]. MATRIX is a phase I/II study (NCT02358161) showing the safety and efficacy of combination therapy including Sonidegib, gemcitabine, and nab-paclitaxel in participants with metastatic PC. The scientists identified that the maximum tolerated dose (MTD) of LDE225 is 200 mg once daily, and co-administered with gemcitabine 1000 mg / m 2 and nab-paclitaxel 125 mg / m 2 . They further found that 13% of patients had a partial response (PR), 58% of patients had stable disease (SD), and 29% of patients showed progressive disease (PD). The median OS was 6 months. Moreover, six treatment-related grade 4 adverse events (AEs) and three grade 5 AEs were observed in phase 2 [ 332 ]. Moreover, CXCR2 signaling was found to be involved in iCAF formation and CAF to myCAF conversion. Studies previously done by researchers suggested that blockade of the CXCR2 axis decreased tumor angiogenesis and PDAC invasion. Combined inhibition of CXCR2 and CSF1R can reduce granulocyte intratumor infiltration and exhibit strong anti-tumor efficacy [ 128 , 333 , 334 , 335 ]. Saxena, S. and colleagues revealed that diverse CXCR2 ligands can potentially become diagnostic markers for PC patients [ 336 ].
Targeting CAF-mediated immunosuppression
In PDAC, CTL and NK T cells are selectively excluded, allowing cancer cells to evade immune surveillance. This makes increasing CTL aggregation or combating the immunosuppressive TME a therapeutic strategy. Based on the use of an anti-mesothelin monoclonal antibody (MSLN Ab) can inhibit the transformation of mesothelial cells into fibroblasts in mouse models of liver fibrosis, as well as the transformation relationship between apCAF and mesothelial cells, researchers found that using MSLN Ab can diminish mesothelial cell to apCAF transition in vitro and in vivo . Furthermore, this treatment drastically lowered tumor weight and reduced iTreg abundance while increasing the percentage of CD8 + T cells in the meantime [ 183 , 337 ]. Hypoxia-inducible factors (HIFs), first discovered by Semenza and Wang in 1992, are the predominant regulators of the response to hypoxia, and HIF-2α was found to be indispensable for Treg functions. A recent study observed that deleting CAF-HIF2 in mice hindered the intratumoral recruitment of M2 macrophages and Tregs, which were closely associated with immune suppression. Furthermore, after utilizing PT2399, a HIF-2α antagonist used previously to target renal cell carcinoma, tumor responses to immunotherapy were enhanced [ 338 , 339 , 340 , 341 ]. Proline isomerase Pin1 was found overexpressed both in cancer cells and CAFs, which was correlated with the immunosuppressive TME and poor OS, and Pin1 inhibitor can increase the infiltration of CD8 + CTLs, reduce immunosuppressive cells, and may expand the benefits of chemotherapy in GDA and KPC mice [ 342 ]. Notably, Liu, J. and colleagues designed a DNA-barcoded micellular system (DMS) functionalized with CAF-targeting anti-FAP-α antibodies (antiCAFs-DMS) that could deliver AG17724, which was a Pin1 inhibitor directionally to CAFs. Furthermore, DNA aptamer was introduced to induce CD8 + T cell infiltration, forming the anti-CAFs-DMS-AptT. Anti-CAFs-DMS-AptT was demonstrated to eliminate established tumors and alter or regulate TME in subcutaneous and orthotopic PC models [ 343 ].
Targeting tumor stroma
Hyaluronan (HA) is a glycosaminoglycan (GAG), and a significant component of normal ECM overexpressed in several solid malignancies [ 344 ]. HA and collagen collaborate to promote the accumulation of substantial stress (pressure from solid tissue components), which compresses tumor blood vessels [ 345 , 346 ]. Losartan has been shown to reduce TGF-β-mediated activation of CAFs, reduce the development of desmoplastic tissue components like hyaluronan and collagen produced by CAFs, and increase drug delivery and the effectiveness of immunotherapy [ 347 ]. Losartan and other traditional chemotherapy drugs in treating PC are undergoing clinical trials. In a phase II clinical trial that included patients with local advanced PC, neoadjuvant therapy with FOLFIRINOX combined with losartan and chemoradiotherapy demonstrated a high R0 resection rate and prolonged total survival rates [ 348 ]. Moreover, enzymic depletion of HA via pegylated recombinant human hyaluronidase (PEGPH20) has attracted researchers’ attention. Shreds of evidence from preclinical studies in PDA mice models demonstrated that PEGPH-mediated HA depletion could decrease interstitial fluid pressure (IFP), improve vascular perfusion, and elevate chemotherapeutic delivery [ 345 , 349 ]. A phase Ib clinical trial (NCT01453153) enrolled 28 participants with stage IV previously untreated PDAC demonstrated that PEGPH20, combined with gemcitabine, has shown desirable tolerance and may have therapeutic benefits in patients with advanced PC. The PR rate after treatment of PEGPH20 was 35.7%, which was higher than the ORR rate using gemcitabine alone (7–13%), and the treatment-emergent AEs was 96.4% [ 350 ]. Additional clinical trials, including phase II and phase III, were conducted to investigate the value of PEGPH20 plus standard chemotherapy regimens (FOLFIRINOX and AG) in treating metastatic PC [ 351 , 352 , 353 ]. However, an early phase 1 study of PEGPH20 and Avelumab to treat chemotherapy-resistant PC was terminated for an unknown reason (NCT03481920). Some abovementioned mechanisms are summarized in Fig. 4 .
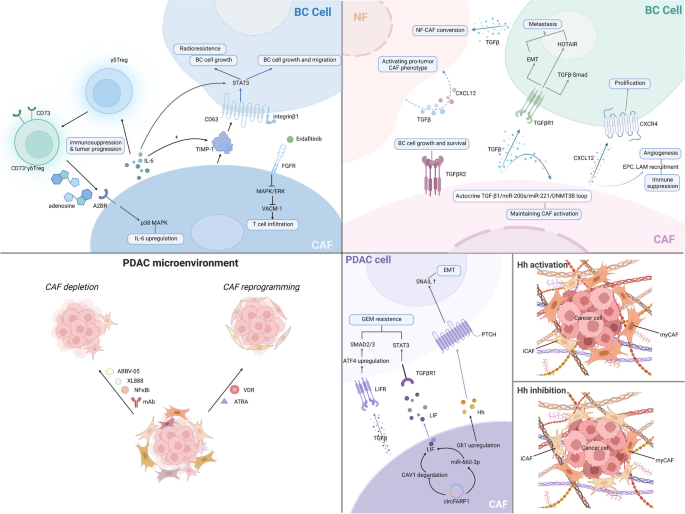
Schematic diagram of the interaction of CAF with cells in BC and PDAC TME. The IL6-adenosine loop potentiates immunosuppression and BC progression, and the TIMP-1/CD63/integrin β1/STAT3 loop is associated with BC cell growth. Erdafitinib promotes T lymphocyte infiltration via inhibiting MARK/ERK signaling. Moreover, CAF-secreted TGF-β1 activates the transcription of HOTAIR to promote BC cell metastasis; the autocrine TGF-β1/miR-200 s/miR-221/DNMT3B loop maintains CAF activity and promotes BC progression. CAF-secreted CXCL12 favors BC cell proliferation and EPC, LAM recruitment. In PDAC, CAF reduction can be achieved by depleting or reprogramming CAF. CAF-derived circFARP1 and TGF-β can both lead to gemcitabine resistance in PDAC cells, and CAF-secreted Hh promotes EMT via upregulating SNAIL transcription. In the end, Hh inhibition changes the proportion of CAF phenotypes in PDAC TME. By Biorender
Lung cancer
Only about 20 percent of lung cancer (LC) patients survive for five years. LC was the deadliest in 2019, claiming approximately 2 million lives worldwide. The most common LC was NSCLC, known for its uncomplicated metastasis and drug resistance, but the mechanism is still unclear [ 354 ]. But in recent years, with the increase in awareness of CAF, strategies targeting CAF have proven effective in LC treatment.
Targeting CAFs via biomarkers
The CAR-T strategy has made noticeable progress in LC treatment. According to some studies, FAP can be genetically engineered to become a viable target for CAR. In preclinical studies, FAP-specific CARs were developed to redirect T cells to FAP-positive CAFs. These T cells can form a specific immune attack against FAP + CAFs, with concomitant antitumor efficacy and no apparent signs of toxicity [ 355 , 356 ]. Prior studies have shown that FAP-CAR T cell therapy in human LC xenografts and homologous mouse PC models can reduce tumor vascular density, restrain desmoplasia, and grow native PC [ 357 ]. Lately, a phase I clinical trial (NCT01722149) enrolled four patients with metastatic pleural mesothelioma (MPM) using FAP targeting CAR T-cells (CART-FAP) have been reported safe, with major SAEs including upper respiratory infection and thromboembolic event. Intra-pleural injection of single CART-FAP in MPM patients is safe and increases proinflammatory cytokines levels in patients’ sera. However, due to the small number of patients enrolled, the impact of this treatment on patient outcomes could not be evaluated and entailed further investigation [ 358 ]. More recently, the combination of Nectin4-targeted CAR-T (Nectin4-7.19 CAR-T) and FAP-targeted CAR-T (FAP-12 CAR-T) cells was demonstrated by Li, F., and colleagues to exterminate lung metastasis in the NSG mouse model. Furthermore, the radiolabeled FAP inhibitors applied to PET imaging can potentially monitor therapeutic response to FAP-targeted CAR T-cell therapy, reducing the limitations of CAR T-cell therapy. In addition, the therapeutic effect of CART is also gradually established in HCC and glioblastoma [ 359 , 360 , 361 , 362 , 363 ]. Unfortunately, it has been observed that using FAP-targeted CAR T cells to target subcutaneous tumors in mice led to deadly myelotoxicity and cachexia [ 224 ].
Targeting the signaling pathways of CAFs
The TGF-β signaling pathway promotes EMT through the secretion of IL-6 in NSCLC. It induces tumor tissue remodeling by transforming entities to acinar in LC cells, affecting the histological pattern of lung adenocarcinoma (LUAD) [ 364 , 365 ]. CUDC-907 is a dual inhibitor of the HDAC and PI3K/AKT pathways that inhibited the proliferation and differentiation of CAF, which was induced by TGF-β1, as well as the collagen expression. In a phase 1b/2 study (NCT02423343), scientists enrolled 41 participants who suffered from advanced solid tumors and recurrent NSCLC or HCC. In phase 1b, patients were divided into four cohorts receiving Galunisertib orally at the dose of 50 mg once daily, 50 mg twice daily, 80 mg twice daily, and 150 mg twice daily on Day 1 through Day 14 of each 4-week cycle combined with 3 mg/kg nivolumab given intravenously until discontinuation criteria are met. Researchers identified that the MTD of Galunisertib was 300 mg. In phase 2, patients with NSCLC or HCC were administered Galunisertib 150 mg twice daily on Day 1 through Day 14 of each 4-week cycle combined with 3 mg/kg nivolumab given intravenously. The results showed that when administered with Galunisertib, no patients were found to have anti-Nivolumab antibodies. The median PFS was 5.26 months, and the ORR was 24% in patients with NSCLC. The immunogenic effects of radiotherapy (RT) may be counteracted by avoidance mechanisms in TME, including induction of angiogenic factor secretion and CAF activation. At the same time, BA can reverse radiotherapy-induced CAF activation and fibrosis. Altogether, Y, L., and colleagues suggested that BA + RT (BART) combinations potentially eradicated drug-resistant tumors while preserving normal tissue [ 366 ]. Moreover, Shi, X., and colleagues revealed that TGF-β inhibitor LY2109761 decreased the expansion of squamous cell carcinoma (SCC) CAFs in the lung in vivo, and another TGF-β inhibitor, LY2157299, can inhibit the formation and invasion of CSC-CAFs co-cultured spheres in vitro [ 367 ].
P62 has been found to be associated with lung adenocarcinoma progression. Inhibition of autophagy with hydroxychloroquine (HCQ) reduced CAF activation and TGFβ production, thereby hindering tumor growth. Mechanistically, p62-induced autophagy upregulates the expression of nuclear factor erythroid 2 correlated factors 2 (Nrf2) and activated transcription factor 6 (ATF6) to promote CAF activation and pharmacological inhibition of the Nrf2-ATF6 pathway can completely block CAF activation [ 368 ]. In addition, CAF-derived SDF-1 induced the EMT of LUAD cells via CXCR4/β-catenin/ PPARδ signaling. Thus, using the β-catenin inhibitor XAV-939 and PPARδ inhibitor GSK3787 to target the CXCR4 β-catenin/ PPARδ cascade attenuated EMT, which might serve as a potential therapy for LC treatment [ 369 ]. Moreover, the third-generation EGFR inhibitor osimertinib treats tyrosine kinase inhibitor (TKI)-resistant NSCLC, and most patients eventually become osimertinib-resistant. Studies have shown that the MEK inhibitor trametinib can eliminate the high expression of FAP and excessive secretion of IL-6 in osimertinib-resistant cells by inhibiting the MEK / ERK / miR-21 axis. In the xenograft model, osimertinib and trametinib combination therapy had a significant growth-inhibiting effect on osimertinib-resistant NSCLC tumors [ 370 ]. Li, H. et al . also unraveled that the intracellular accumulation of reactive oxygen species (ROS) can activate the STAT3 pathway, allowing senescent fibroblasts to exhibit CAF signatures, thereby expediting the migration of H1299 and A549 LC cells [ 371 ].
Other targets
Metabolic reprogramming is a crucial feature of cancer that allows cancer cells to survive and proliferate wantonly. Recent studies have shown that cancer cells exhibited high glutamine uptake in TME [ 372 , 373 ]. A CAF-specific lncRNA, LINC01614, enhanced glutamine metabolism in LUAD cells by promoting NF-κB activation and upregulating the expression of glutamine transporters SLC38A2 and SLC7A5. Furthermore, the pro-inflammatory factors secreted by cancer cells, such as IL-6 and CXCL10, can upregulate the expression of LINC01614 through a feedforward loop. Liu, T., and colleagues stated that LINC01614 was associated with poor prognosis in patients and that deleting LINC01614 reduced metastasis in NCG mice and zebrafish cancer models, suggesting that targeting particular lncRNA can attenuate glutamine utilization in cancer cells, potentially delaying cancer progression [ 374 ].
TAM was reported to promote CAF formation through MMT, and Smad3 was one of the critical regulators in this process. Macrophage‐lineage CAF and tumor growth were significantly impaired in Lewis lung carcinoma (LCC) mice models after utilizing the SMAD3 inhibitor, SIS3 [ 72 ]. In addition, because SIS3 was identified to promote NK cell cytotoxicity by improving Smad3-mediated inhibition of Ndrg1 transcription, it also made it one of the potential therapeutic strategies for LC [ 375 ]. Besides, research concentrating on reprogramming CAFs has pointed out that when CAFs were exposed to apoptotic cancer cells, apoptotic cancer cells reprogramed CAF through the Notch1-WISP-1 signaling pathway, inhibiting cancer invasion and metastasis. This effect was also demonstrated in mice, where injection of apoptotic 344SQ cells (ApoSQ) inhibited CAF activation, as evidenced by reduced mRNA levels of CAF cytokines [ 376 ].
Colorectal cancer
CRC was the fourth deadliest cancer in the world in 2019. Because its symptoms are usually insidious in the early stages, how to recognize CRC early is an overarching problem to overcome. Currently, the treatment methods for CRC mainly include endoscopic and surgical local resection, palliative chemotherapy, targeted therapy, and immunotherapy [ 377 ]. However, research and drugs targeting CAF are still being established in CRC, unlike other cancers. Several genes associated with CAF are possible therapeutic targets, including COL3A1, JAM3, AEBP1, WNT2, and WNT54 [ 378 , 379 ]. Research on the origins of CAF recently revealed that many ACTA2 CAFs are derived from the proliferation of intestinal pericryptal leptin receptor (Lepr) cells expressing melanoma cell adhesion molecules (MCAM) through lineage tracing. In mouse models, matrix MCAM knockout attenuated colorectal tumoroid growth injected in situ. Therefore, preventing the differentiation of leprosy spectrum CAF or inhibiting the activity of MCAM might be an effective treatment for CRC [ 380 ]. Below, we will elaborate on other approaches to target CAF.
Targeting signaling pathways
Targeting TGF-β pathway strategy is also described in CRC. CAF-derived exosomes boosted stemness of CRC cells via TGF-β signaling, thereby improving radiation resistance, and this effect was attenuated after the use of neutralizing antibodies [ 381 ]. Yang, M., and colleagues revealed that the overexpression of fibronectin leucine-rich transmembrane protein 3 (FLRT3) can restrain EMT, and FLRT3 downregulation is associated with poor prognosis. CAF reduced FLRT3 expression by activating the TGF-β/SMAD4 signaling pathway and enhanced CRC aggressiveness. TGF-β inhibitor LY2109761 can attenuate this effect, reducing the amount of Treg in TME [ 382 ]. Besides, resveratrol (RES) can also suppress EMT through TGF-β1/Smads signaling in CRC [ 383 ]. Intriguingly, resveratrol-loaded liposome (L-RES) reduced α-SMA and IL-6 levels in activated fibroblasts and disrupted crosstalk between CRC cells and CAF to inhibit the function of CAF [ 384 ]. The anti-tumor role of RES was also observed in BC, LC, and PCa [ 385 , 386 , 387 ]. According to Naktubtim, C. and colleagues, YAP regulates CAF transformation associated with F-actin rearrangement, thereby promoting CRC cells' proliferation, migration, invasion, and angiogenesis. The YAP inhibitor verteporfin (VP) can reverse the above reaction. More recently, the IGF2-IGF1R-YAP1 axis was demonstrated to be a prognostic biomarker and therapeutic target for CRC. The expression of IGF2 in CAF was upregulated, while IGF1R was mainly expressed by cancer cells. IGF2 can cause YAP1 to accumulate within the nucleus. However, the cascade activation mediated by IGF2 was dispelled by IGF1R depletion and an IGF1R inhibitor, picropodophyllin (AXL1717). Moreover, the AXL1717 and VP combination therapy showed greater anti-tumor efficacy than PPP alone [ 388 , 389 ]. γ-mangostin (γ-MG), a critical active substance isolated from mangosteen, inhibited the GSK3/β-catenin/CDK6 pathway associated with CRC stemness. Using gMG in xenograft mouse models inhibited tumor growth and overcame CAF-induced 5-fluorouracil resistance [ 390 ]. It is reported that CAF-secreted IL-6/IL-11 can activate STAT3 signaling, further facilitating CRC progression. Correspondingly, inhibiting the activation of the STAT3 pathway in COL1 + CAFs can impair CRC development in the AOM/DSS model [ 391 , 392 ]. Although therapeutic trials using STAT3 inhibitors have demonstrated significant results in certain oncological diseases, a randomized phase 3 trial showed that OS in the patient cohort was not expected when the STAT3 inhibitor napabucasin was used for refractory metastatic CRC [ 393 ].
Targeting CAF-derived exosomes
The exosomal LncRNA LINC00659, originating from CAF, has been shown to promote the proliferation and migration of CRC cells by activating ANXA2 and downregulating the expression of miR-342-3p. In addition, exosomes miR-146a-5p and miR-155-5p were found to have increased expression in CXCR7-overexpressed CRC cells. These two exosomes can be absorbed by CAF, which is conducive to the activation of CAF. Functional studies have shown that activated CAFs highly express inflammatory factors such as IL-6 and CXCL12 to promote the invasion and metastasis of CRC cells. Significantly, miR-146a-5p and miR-155-5p activate CAFs to promote tumor formation and lung metastasis of CRC in vivo in tumor xenograft models [ 394 , 395 ]. Other CAF-derived exosomes such as WEE2-AS1 and circEIF3K have also been reported to potentiate CRC progression via inhibiting the Hippo signaling pathway and activating the miR-214/PD-L1 axis, respectively [ 396 , 397 ]. Furthermore, certain exosomes contributed to CRC angiogenesis, chemoresistance, and radioresistance [ 398 , 399 , 400 ]. Taken together, targeting exosome therapy was suggested to become a promising strategy for CRC treatment. Moreover, CAF-derived lncRNA (CAFDL) can predict the prognosis of LUAD, BC, thyroid cancer, and many other cancers. CAFDL can be used as a risk stratification tool to predict the clinical outcome of CRC [ 401 ]. However, specific drugs targeting exosomes have rarely been reported, so it is urgent to study new agents and verify their clinical safety and feasibility.
Targeting TME and metabolism
Shen, W., and colleagues designed a nanoemulsion combining chemotherapy and gene therapy to simultaneously deliver doxorubicin and small interfering RNAs targeting hepatocyte growth factor (HGF) to reduce ECM deposition, induce CAF apoptosis, and reduce tumor metastasis. The chemotherapy resistance was ameliorated somewhat, and the tumor progression was inhibited [ 402 ]. Another tyrosine kinase inhibitor, NT157, can pharmacologically inhibit IGF-1R and STAT3 signaling. It can target CRC by simultaneously influencing tumor-associated cells and their supportive microenvironment, such as inhibiting the secretion of pro-tumor cytokines [ 403 ]. As mentioned in LC, CAF can also impact the metabolism of CRC. CAF reprograms CRC metabolism by stimulating glycolysis, oxidizing arms of the pentose phosphate pathway (PPP), and inhibiting the tricarboxylic acid cycle. The researchers found that knocking out hexokinase and glucose-6-phosphatase in CAF-treated CRC cells slowed tumor growth. They also proposed that using inhibitors of these two enzymes, including metformin and polydatin, as well as emerging bispecific antibodies and proteolytic targeted mosaicism (PROTACs), could be a potential therapeutic approach [ 404 ]. Additionally, MMP14-expressing CAFs were found to be associated with CRC progression, and patients with high MMP14 CAF/CAF ratios exerted adverse outcomes, which may have prognostic value [ 405 ].
Prostate cancer
The incidence of PCa ranks second in the world among male malignancies. In 2022, the incidence of prostate cancer in the United States has surpassed LC, becoming the most severe malignant tumor that endangers men's health. The treatment methods for PCa mainly include endocrine hormone therapy, radiotherapy, chemotherapy, surgical treatment, emerging immunotherapy, etc. Among them, castration therapy is an imperative treatment, but cancer cells will inevitably become resistant to castration therapy. Studies have reported that CAF has a promoting effect on castration resistance. Coupled with the previously described CAF functions, targeting CAF and its related activities has become a promising treatment. Moreover, some CAF gene signatures such as ACPP, THBS2, TMEM132A, and ZNF467 were established to estimate the survival of PCa patients undergoing radiotherapy [ 406 ].
Targeting CAFs specific biomarkers and signaling pathways
Targeting FAP strategies have also been proposed and applied in PCa. A nanosystem delivering FAP antibodies can inactivate CAF by downregulating the expression of CXCL12 to modulate the tumor microenvironment of PCa [ 407 ]. 68 Ga-FAPI was highly uptaken by PCa cells, so it is used for diagnosis in PET/CT imaging [ 408 , 409 ]. Moreover, Kakarla, M. and colleagues unraveled that CAF showed higher expression of Ephrin B1, B2, and B3 ligands (EFNB1, EFNB2, and EFNB3) compared to NF. It has also been mentioned that high levels of EFNB1 and EFNB3 in benign human prostate stromal cell lines can potentiate tumorigenicity in PCa cells and activate Src family kinases (SFKs) in prostate fibroblasts. SFK inhibitor AZD0530 (Saracatinib) decreased the expression of the CAF marker ɑ-SMA and ECM protein TNC in vitro [ 410 ]. As for CAF-associated signaling pathways, targeting the CXCL12/CXCR4 axis has also shown novel advances in PCa. For instance, the aforesaid AMD3100 can reduce tumor angiogenesis and decrease the migration of tumor endothelial cells (TEC) in vitro [ 411 ]. Alternatively, the inhibition of TGFβ and CXCL12 can overcome the immune suppressive microenvironment. MPSSS, a natural polysaccharide extracted from shiitake mushrooms, disrupted T cell inhibition mediated by CAF via activating TLR4-NF-κB signaling [ 412 ]. Notably, the decreased expression of some CAF biomarkers and TGFβ2 caused by silibinin was observed in PC3 tumors, which attenuated NFs to CAFs conversion, suggesting the therapeutic potential of these natural flavonoid lignans. Again, FOXF2 (Forkhead Box F2) in the prostate stroma reduced the iCAF phenotypic ratio, induced its transformation to myCAF, and downregulated CXCL5, thereby reducing immunosuppressive myeloid cells and enhancing T cell cytotoxicity. Further, increasing the content of Foxf2 sensitized PCa to ICB therapy [ 413 ]. The gene set variation analysis by Zhai, X., and colleagues indicated that pathways like PPAR, GnRH, and mTOR are significantly correlated with PCa and might become targeted landmarks [ 414 ].
Targeting CAF-induced castration resistance
Castration therapy is currently one of the mainstream treatments for PCa. Still, castration resistance is also a troublesome problem, and emerging evidence validates that CAF contributes to resistance via diverse mechanisms. When castration-resistant PCa CSC and castration-resistant prostate cancer CAF (CRPCAF) were Adisetiyo, H. and colleagues observed co-cultured, more aggressive tumorigenesis. CRPCAF had a far more powerful ability to support organoid/tumor formation than androgen-dependent PCa CAF in vivo and in vitro, suggesting that CRPCAF can improve the stemmatic and tumorigenic properties of CSCs [ 415 ]. Androgen receptor (AR) is normally expressed in prostate epithelial cells, PCa cells, and prostate fibroblasts. It has been reported that AR inactivation upregulated LIM domain only 2 (LMO2) expression in prostate CAF, and paracrine IL-11 and FGF-9 can activate pathways such as STAT3, AKT, etc., which subsequently activated AR in PCa cells, leading to castration resistance. Correspondingly, the use of neutralizing antibodies or pathway blockade exhibited a decrease in AR activation [ 416 ]. Research showed that CD105 CAF could mediate neuroendocrine epithelial differentiation and castration resistance in prostate tumors in a paracrine manner [ 417 ]. Neuromodulator 1 (NRG1) secreted by CAF can promote tumor cell resistance to antiandrogen therapy by activating HER3. The use of pharmacological inhibition of the NRG1-HER3 pathway with YW538.24.71 (NRG1-neutralizing antibody), AMG888 (HER3-blocking antibody), and neratinib (HER2 kinase inhibitor) significantly inhibited the growth of CWR22Pc tumor, a patient-derived xenograft model. It restored their responsiveness to anti-androgenic therapy [ 418 , 419 ]. Glucosamine secreted by CAF has increased O-GlcNAcylation in PCa cells, thereby promoting Elk1-mediated HSD3B1 transcription. The expression of the vital enzyme 3β-Hydroxysteroid dehydrogenase-1 (3βHSD1) of the extragonadal androgen synthesis pathway, which HSD3B1 encoded was increased. As a result, the synthesis of androgens in tumors can be upregulated to form castration resistance. Blocking Elk1 inhibited CAF-induced androgen biosynthesis in vivo [ 420 ].
Targeting metabolism and TME
One of the energy metabolism characteristics of tumor cells was identified as heavy dependence on glycolysis and the production of large amounts of lactic acid, which is deposited to promote tumor progression by multiple mechanisms. In PCa, studies determined that CAF predominantly secretes lactate, and tumor cells store lipids into lipid droplets to support mitochondrial metabolism. Inhibition of BET with I-BET762 (Molibresib) to target histone acetylation interfered with lactate-dependent lipid metabolism and could impede prostate cancer growth and metastasis in mice [ 421 , 422 , 423 ]. Furthermore, an open-label phase I trial (NCT01587703) of Molibresib has been conducted in patients with castration-resistant prostate cancer (CRPC), CRC, NSCLC, and other cancers. The study consisted of two parts. Part 1 of the study was a dose escalation establishing the recommended dose for part 2, and part 2 assessed the safety, pharmacokinetics, and pharmacodynamics of the recommended dose. It is indicated in part 1 that oral administration of Molibresib 80 mg once daily was the recommended phase 2 dose. In part 2, the results showed that only one participant with CRPC completed the study. 84 patients (82%) in all cohorts suffered from grade 3/4 AEs, mostly thrombocytopenia (43%) and anemia (21%). Only one confirmed PR was reported in CRPC. In conclusion, treatment with Molibresib 75 mg once daily was tolerable but may require dose interruptions. And the anti-tumor efficacy entailed further investigation. Alternatively, Neuwirt, H. et al . revealed that CAF could boost cholesterol and steroid biosynthesis levels in PCa cells by highly upregulating the expression of HMGCS2 and AKR1C3 to promote androgen receptor-targeted therapy resistance in PCa. Notably, the dual inhibition of cholesterol and steroid synthesis with simvastatin and AKR1C3 inhibitors demonstrated significant tumor growth inhibition [ 424 ].
In 2017, the overexpression of LOXL2 in PCa tissues was confirmed. Two years later, Nguyen EV and colleagues found through proteomic analysis that proteins related to cell adhesion and extracellular matrix were significantly enriched in CAF, including LOXL2, discoidin domain-containing receptor 2 (DDR2), etc., compared with normal fibroblasts. Of note, using LOXL2 inhibitors D-penicillamine and PXS-S2A can hinder CAF migration and ECM alignment [ 145 , 425 ]. Besides, LOXL2 knockdown enhanced castration-resistant PCa radiosensitivity in vitro and in vivo conditions and provided insight for solving the resistance of PCa radiotherapy [ 426 ]. Additionally, a previous study has shown interactions between M2 macrophages and CAF. On one hand, M2 macrophages promoted EMT conversion; on the other hand, CAF secreted SDF-1 to recruit monocytes and facilitated their differentiation into M2 macrophages. G protein-coupled receptor 30 (GPR30) is an estrogen receptor highly expressed in prostate CAFs. Knocking it out hinders macrophage infiltration and M2 polarization, adversely affecting PCa invasion [ 427 , 428 ]. Subsequently, BXCL701, a small molecule inhibitor of dipeptidyl peptidase (DPP) that can trigger inflammation within TME and increase immunotherapy responsiveness, was recently studied in a phase 1b/2 study (NCT03910660) that enrolled 95 participants to evaluate the efficacy and safety of BXCL701 oral, monotherapy, and in combination with pembrolizumab (PEMBRO) in patients with mCRPC. The trial was divided into three parts, the first part described above, and the second part divided patients into two cohorts by cancer type: small cell neuroendocrine prostate cancer (SCNC) and adenocarcinoma phenotype to observe the composite response rate of BXCL701 + PEMBRO. Phase 2b of the study will only enroll patients with histological subtypes showing preliminary evidence in Phase 2a, assessing response rates in patients treated with BXCL701 + PEMBRO and BXCL701 monotherapy. However, the study is now active, not recruiting. EXPEL PANC was an ongoing clinical trial (NCT05558982) testing the same drug combination BXCL701 + PEMBRO in patients with metastatic PDAC. The research was expected to improve patients’ progression-free survival at 18 weeks, but the result has not been published.
Melanoma, malignant from melanocytes, is the deadliest type of skin cancer, usually triggered by ultraviolet, trauma, etc. Melanoma is expected in the skin but is not limited to the skin, it can also occur in the conjunctiva, oral cavity, etc. FDA-approved immunotherapy like anti-PD-1 therapy and targeted drugs vimofenib have brought hope to melanoma patients, significantly improving their five-year survival rates [ 429 , 430 ]. Targeting CAF has become a promising treatment strategy for melanoma as well. Studies have pointed out that because stromal fibroblasts and CAFs have higher genetic stability, they are less likely to develop drug resistance than malignant cells.
Targeting CAFs-related proteins
To date, conventional therapies against CAF have not been widely reported in melanoma. NRG1, highly expressed in fibroblasts and CAF, is a ligand for ErbB3, and fibroblast-derived NRG1 can attenuate the effect of RAF inhibitors on melanoma cells. Targeting the ErbB3/ErbB2 pathway with neutralizing antibodies seribantumab (MM-121) and pertuzumab counteracted the protective effects of CAF and improved melanoma cell drug responsiveness [ 431 ]. Seribantumab has been investigated in 44 patients with advanced solid tumors in a phase 1 clinical trial (NCT00734305). The participants were divided into 6 cohorts and received seribantumab intravenously once a week for a maximum of 47 weeks at the beginning dose of 3.2 mg/kg, and the dose escalated in separate cohorts from 6 mg/kg, 10 mg/kg, 15 mg/kg, 20 mg/kg, to the highest scheduled testing dose at 40 mg/kg one-time loading dose on cycle 1, week 1 followed by 20 mg/kg maintenance doses. ORR is 0 for the 6 cohorts, and the incidence of serious AEs and other AEs was 32.56% and 100%, respectively. The most reported AEs were nausea (46.51%) and fatigue (48.84%). In another phase 2 study (NCT04383210), researchers aimed to assess the ORR of seribantumab in patients with recurrent, locally advanced, or metastatic solid tumors, which harbor the NRG1 gene fusion, but the outcomes remain unknown as well. It is believed that the β-catenin in Wnt/β-catenin signaling might be associated with early melanoma. In a Col1α2-CreER mouse model in which β-catenin was knocked out from dermal fibroblasts, researchers observed a decrease in chemokines and ECM protein production. They proposed that dermal fibroblasts acted as a physical barrier and exerted anti-tumor function before shifting to CAFs. To further substantiate the role of β-catenin in CAF, a study by Zhou, L. and colleagues observed that the β-catenin pathway blockade in CAF magnificently inhibited the proliferation of melanoma in a PTEN-deficient mouse model activated by BRAF and the EMT process of melanoma cells was also suppressed. More recently, BRAF inhibitors induced β-catenin accumulation in CAF, stimulated the secretion of the downstream effector POSTN of β-catenin signaling, and finally activated ERK signaling to allow melanoma cells to continue proliferating in the presence of BRAFi and MEKi, suggesting that explicitly targeting POSTN may be one of the promising options [ 432 , 433 , 434 ]. On the contrary, some novel mechanisms have been discovered that may guide the establishment of strategies targeting CAF. According to studies from Papaccio, F., CAF can affect melanoma cell migration and protect melanoma cells from paclitaxel-induced apoptosis [ 435 ].
Since exosome is a vector for crosstalk between CAFs and melanoma cells, several studies have concentrated on dissecting its inherent mechanism [ 436 , 437 ]. It was reported that miR-155 derived from melanoma cells could inhibit the expression of tumor suppressor gene SOCS1 in CAF, activate the JAK2/STAT3 signaling pathway, and trigger the transformation of CAF to proangiogenic type. Interestingly, the single inhibition of exosomal miR-155 could not restore the original angiogenic factor levels, indicating that other factors synergistically promoted the proangiogenic conversion of CAF, which remained to be explored [ 438 ]. Furthermore, Dror, S. and colleagues isolated 5 microRNAs from mature melanosomes, of which the most abundant miR-211 was shown to be transported from melanocytes to fibroblasts through melanosomes, causing phenotypic changes in co-transplanted mouse models, that is, reprogramming NF to CAF. In terms of mechanism, the response to promote collagen contraction and pro-inflammatory factor secretion was achieved by downregulating the expression of tumor suppressor IGF2R and activating the MAPK signaling pathway. Of note, using the p38 inhibitor SB202190 (FHPI) reduced the secretion of melanosomes and miR-211, and the presence of the ERK (a marker for MAPK signaling activation) inhibitor U0126 also eliminated the effect of miR-211 on NF [ 439 , 440 , 441 ]. Notwithstanding, none of these inhibitors have moved into clinical trials yet.
In addition, a 2021 study found that neutrophils in mouse PC and melanoma models often aggregated in CAF-rich regions, creating extracellular traps (NETs). Specifically, CAF drove tumor-induced NETs (t-NETs) formation through the ROS pathway and amyloid β, and the interaction between CAFs and NETs was reciprocal. The PAD4 inhibitor GSK484 in vivo to inhibit histone citrullination in the ROS pathway in a melanoma mouse model was observed to impair tumor growth. In addition, BACE inhibitors can suppress amyloid β production, thereby hindering tumor development. The blockade of CD11b, a possible receptor of amyloid β on the surface of neutrophils, essentially eliminated NETosis. Eventually, they proposed that the amyloid β-NET axis behaved analogically in human PC and melanoma as in mice, which was associated with poor survival [ 442 ]. This study defined a novel mechanism between CAFs and NETs. It provided three potentially valid targets to target the amyloid β-NET axis, paving the way for further investigation in humans. Some abovementioned mechanisms are summarized in Fig. 5 .
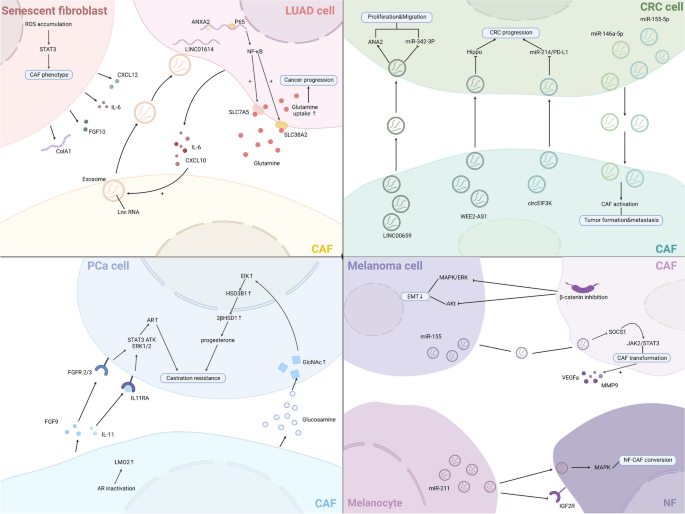
Schematic diagram of the interaction of CAF with cells in LC, CRC, PCa, and Melanoma TME. CAF-secreted exosome LINC01614 activates the NF-κB signaling pathway, thus upregulating the glutamine transporters SLC38A2 and SLC7A5 to promote glutamine uptake. In CRC, the exosomal LINC00659, WEE2-AS1, and circEIF3K from CAF enhance CRC progression through various mechanisms, and the miR-146a-5p and miR-155-5p from CRC cells activate CAF. Moreover, through FGF9, IL-11, and glucosamine secretion, CAF expedites castration resistance in PCa cells. At last, inhibition of β-catenin in CAFs downregulates AKT and MAPK/ERK signaling and blocks EMT in Braf V600E ; Pten lox5/lox5 melanoma. Melanoma cell-secreted miR-155 and melanocyte-secreted miR-211 promote CAF transformation and NF-CAF transition respectively. By Biorender

Other cancers
Irrespective of the aforementioned cancers, novel mechanisms, and protocols targeting CAF have also prospered in other cancer types. A cytokine named cardiotrophin-like cytokine factor 1 (CLCF1), derived from CAF, can promote the secretion of CXCL6 and TGF-β in HCC cells in an Akt/ERK1/2-STAT3 pathway-dependent manner. These upregulated chemokines then worked synergistically to expedite stem properties of tumor cells and tumor-associated neutrophil polarization, and TGF-β was also associated with NET formation. This study also demonstrated a positive feedback effect between CXCL6, TGF-β, and CLCF1 by activating the ERK1/2 pathway in CAF. Correspondingly, researchers suggested that selective inhibition of CLCF1/CNTFR (ciliary neurotrophic factor receptor) or ERK1/2 in mouse models precluded crosstalk between CAFs and HCC cells, which might become a potential treatment for HCC patients since up-regulation of CLCF1-CXCL6/ TGF-β cascade in HCC patient samples was pertaining to poor prognosis [ 443 ]. A lipid with endogenous anti-inflammatory effects, Resolvin, has been shown to impede tumor progression and increase therapeutic efficacy. In 2019, Sun, L. et al . demonstrated that Resolvin D1 (RvD1) impaired CAFs-induced tumor stemness, EMT of HCC cells, and production of cartilage oligomeric matrix protein (COMP), which had an apparent pro-tumor function. Later, RvD1 was shown to influence monocyte recruitment and the activity of tumor-associated neutrophils, reducing the proliferation of HPV-positive cancer cells in mice and humans in vivo and in vitro. Therefore, activating RvD1 or exogenous supplementation may have effective therapeutic outcomes [ 444 , 445 , 446 ]. Additionally, the overexpression of SPP1 was found in patients who did not respond to sorafenib, and patients with SPP1‐related gene expression displayed some adverse outcomes. Eun, J. W. and colleagues revealed that CAF-secreted SPP1 could reinforce HCC TKI resistance via activating RAF/MAPK and PI3K/AKT/mTOR signaling and favors EMT as well, indicating that SPP1 blockade may have clinical value [ 447 ].
In GC, HSF1-mediated upregulated inhibin subunit beta A (INHBA) and Thbs2 are secreted from CAF via extracellular vesicles, promoting aggressive GC phenotype and progression [ 448 ]. More importantly, there is much evidence that CAF is highly correlated with drug resistance in GC. CAF has been demonstrated to confer 5-fluorouracil (5-FU) resistance to GC cells by expressing neuropilin 2 (NRP2), eventually activating the Hippo pathway and alternatively activating JAK/STAT3 in cancer cells via secreting cytokines. Ham, I.-H. and colleagues have claimed that curcumin can inhibit the JAK2/STAT3 pathway activated in GC cells. In xenograft models, curcumin synergistically inhibits the growth of GC with 5-FU, suggesting that this natural substance extracted from ginger has a prominent anti-tumor ability [ 449 , 450 ]. As mentioned earlier, the stiffened ECM induced by CAF also favored chemoresistance. According to Lu, Y., the overexpression of calponin1 (CNN1) in CAF enhanced the contraction of CAF, leading to stromal sclerosis, and the hardened stroma acted on GC cells, thereby activating YAP signaling and causing chemotherapy resistance [ 451 ]. Besides, it has been suggested that the redundant matrix was associated with immunosuppression. A designed drug combination comprising dual PDGFRα/β suppression by regorafenib and anti-PD-1 therapy exhibited good efficacy in treating fibrotic tumors since blocking PDGFRα/β can invert the immunosuppression in murine GAN-KP tumors. Multikinase inhibitor regorafenib itself was demonstrated to decrease CAF-secreted chemokines, thus modulating TME to a less immunosuppressive state [ 452 ]. A phase 2 study (NCT01913639) enrolled 39 participants with unresectable or metastatic esophagogastric cancer intended to evaluate the effects and safety of regorafenib with chemotherapy regime (FOLFOX) including 5-Fluorouraci, Leucovorin, and Oxaliplatin. The results showed that 53% of the participants showed PFS, 54% of the participants were with CR or PR, and the median OS was 14.2 months. 17 patients (43.59%) suffered from serious AEs such as abdominal pain (23.08%) and dyspnea (10.26%), and 36 patients (92.31%) suffered from other AEs, mostly fatigue (79.49%), anemia (71.79%), nausea (58.97%), and peripheral sensory neuropathy (69.23%). Another phase 3 study (NCT04879368) evaluating the effectiveness of regorafenib and nivolumab combination compared with the standard chemotherapy (Docetaxel, Paclitaxel, Irinotecan, and Trifluridine/Tipracil) in prolonging the OS in a broad group of 450 participants with advanced gastro-oesophageal cancer (AGOC) is now recruiting. In a CAF-conditioned medium (CAF-CM), CAF-secreted midkine (MK) can activate PI3K/AKT signaling via upregulating lncRNA ST7-AS1 expression in GC cell, ultimately enhancing EMT and cisplatin resistance. Similar results that MK affected xenograft tumor growth and chemotherapy resistance via ST7-AS1/AKT/EMT axis were verified in vivo [ 453 ]. Thus, targeting the interactions between CAF and drug resistance might become a therapeutic option.
Bladder cancer is the most common malignancy of the urinary system. ScRNA-seq defined an interferon-regulated subpopulation of bladder cancer CAF, featured by overexpression of the urea transporter SLC14A1. Cohort studies have shown that SLC14A1-positive CAF correlates with poor chemotherapy response and prognosis in clinical patients. Mechanistically, SLC14A1-positive CAFs promoted tumor stemness via the WNT5A/β-catenin pathway, and the cGAS-STING pathway maintained this particular phenotype. The tumor-promoting effect of this CAF was remarkably weakened after the use of an inhibitor of STING or cGAS to suppress this pathway [ 454 ]. Combined therapy has been described in bladder cancer as well. The efficacy of dual suppression of TGF-β and PD-1 pathway utilizing Vactosertib (TEW-7197) and Durvalumab has been investigated in an open-labeled phase II study (NCT04064190). The study is not recruiting yet, and it is anticipated that a total of 48 patients will be enrolled to evaluate whether the cooperation of Vactosertib and Durvalumab can increase patients’ ORR. Durvalumab will be administered with the standard regimen of 1500 mg intravenously every four weeks. Vactosertib will be administered at a dose of 300 mg orally twice a day for 5 days per week, and all treatments will be administered for up to two years. Notably, the aforementioned exosome miR-146a-5p has recently been revealed to have therapeutic potential in bladder cancer and may be highly correlated with urothelial bladder cancer recurrence, as upregulated miR-146a-5p can promote cancer stemness as well as chemotherapy resistance [ 455 ].
Due to the lack of fibroblasts in the central nervous system, it has been suggested that there is no CAF in glioblastoma. Therefore, research reports on glioma CAF are extremely rare. However, recent studies using spatial transcriptomics confirmed the existence of CAF in human glioblastoma and revealed the link between CAF and glioblastoma stem cell (GSC) [ 456 ]. Moreover, the TSP-4 secreted by CAF can improve the transcription level of HSF1 in glioblastoma cells and upregulate lncRNA DLEU1 to confer glioblastoma ferroptosis resistance [ 160 ]. Some of the previously mentioned agents are effective in animal glioma models. AMD3100 encapsulated in synthetic protein nanoparticles (AMD3100-SPNPs) has been validated to diminish glioma growth and restore radiation sensitivity in GBM mouse models in vivo [ 457 ]. Again, NT157 has been found to inhibit glioma cell proliferation and sensitize glioma cells to apoptosis induced by tumor necrosis factor-related apoptosis-induced ligand (TRAIL) via DR5 upregulation [ 458 ]. However, their effects on human glioma have yet to be demonstrated.
Progression in clinical trials of targeted CAF
Although animal experiments still dominate research on CAFs, some clinical trials have shown unsatisfactory results, which may be related to the complex heterogeneity of CAFs. There are still some clinical trials underway, as previously reported. We highlight recent advances in CAF-related clinical trials in multiple cancers in Table 3 . These clinical trials include not only those targeting CAF-expressed markers but also those targeting CAF-related pathways.
Conclusion and future perspectives
The relationship between tumors and TME is like fish and water, in which TME greatly facilitates the proliferation and progression of tumors. Since CAF is an integral part of TME, CAF-targeted therapy has significantly progressed in recent years. The advent of single-cell sequencing and spatial single-cell sequencing has made it possible to analyze specific CAF biomarkers and identify CAF subpopulations. These new technologies ensure the rapid exploration of CAF-specific markers and the development of CAF-specific diagnostic or prognostic protocols. Swift advances in CAF biology have laid a solid foundation for developing novel therapeutic strategies targeting these cells in cancer therapy. However, it is indisputable that CAF has exhibited the juxtaposition of tumor-promoting and tumor-repressing functions. The inherent phenotypic and functional heterogeneity of CAFs may arise from their different cellular origins and thus requires wary consideration when designing CAF-targeted tumor therapies. CAF-targeted therapeutic strategies targeting surface markers, relevant effector molecules, their associated signaling pathways and restricting ECM remodeling have been developed. The main objectives of these approaches are direct or tangential depletion of CAFs, reduction or elimination of their immunosuppressive and tumor-promoting properties, and normalization or reprogramming of CAFs to a quiescent state.
Currently, CAF-based therapeutic strategies are mainly developed in breast cancer, pancreatic cancer, lung cancer, colorectal cancer, prostate cancer, and melanoma. The efficacy of CAF-based therapeutic strategies is expected to be further validated in more cancers. With ongoing research into the molecular mechanisms underlying CAF pathology, many drugs targeting critical regulators of CAF are undergoing clinical and preclinical evaluation. However, it can be concluded that most are phase II clinical trials, and only a few entered phase III clinical trials. Besides, tumor clinical trials directly targeting CAFs still are urgently needed and should be improved.
Of note, targeting CAF strategies has faced several obstacles, including the shortage of CAF-specific biomarkers and their functional heterogeneity. Some studies have unraveled that inhibition of CAF function alone appears to directly inhibit tumor growth, possibly because inhibition of promotional CAF function outweighs inhibition of inhibitory CAF function, as there is also evidence that some CAF subtypes promote tumor development when inhibited. Given that apCAFs are involved in anti-tumor immunity, the mere elimination of CAF presumably triggers serious, unpredictable biological consequences and could end up in crisis. Moreover, an experiment performed in a PDAC mouse model showed that while depletion of αSMA + myofibroblasts reduced fibrosis, an increase in tumor invasion was observed, and gemcitabine did not improve efficacy as a result, which led to diminished animal survival [ 101 ]. Thus, due to technological advances such as single-cell sequencing and novel biomaterials for cell-type-specific delivery, selective elimination of tumor-promoting CAF subpopulations or reversal of their tumor-promoting activity may become a strategy that can be used alone or in combination with other oncology therapies.
Other strategies are proposed in this scenario except for accurately targeting CAF subsets. Targeting transcription through cis-regulatory elements (CREs), such as promoters, enhancers, etc., has been presented as a possible method to target CAF. The use of CAFs as an alternate vector for administering anti-fibroblastic tumor medications has been investigated. Additionally, recent research has shown that fibroblasts have drug-clearing mechanisms. Due to this mechanism, CAFs take up gemcitabine more rapidly than pancreatic tumor cells, implying that targeting metabolic processes rather than removing CAFs altogether may modify the matrix and improve the bioavailability of chemotherapeutic agents in tumors. More importantly, although CAFs are attractive targets in tumors, targeting CAFs may also elicit unpredictable multifaceted stromal responses in the TME that may be patient-heterogeneous due to the complex intercellular interactions involving CAFs in TME. For instance, CAF interacts with tumor-infiltrating immune cells, including TAM, mast cell, NK, and DC, and immune components, including chemokines, cytokines, and effector molecules, to form an immunosuppressive TME. It is expected that therapeutic strategies targeting the cellular interaction between CAF and tumor-infiltrating immune cells could be efficient as well.
Eventually, the aforementioned studies proved that different subtypes of CAF can be converted into each other. This concept was recently confirmed by scientists in PDAC. Synthetic retinol Am80 impeded tissue sclerosis by preventing fiber cross-linking, thereby improving drug delivery efficiency, which was pertaining to the increased expression of the inhibitory biomarker Meflin in CAF, making it more of a tumor suppressor than a tumor promotion [ 459 ]. Therefore, when the treatment for CAF is challenging to carry out, induction of CAF conversion may become an effective treatment modality rather than directly targeting CAF. The synergistic combination of CAF-targeted therapy and other effective treatments, such as chemotherapy, radiotherapy, and immune checkpoint therapy, should also be considered for ultimate tumor eradication.
Availability of data and materials
Not applicable.
Giraldo NA, Sanchez-Salas R, Peske JD, Vano Y, Becht E, Petitprez F, et al. The clinical role of the TME in solid cancer. Br J Cancer. 2019;120:45–53.
PubMed Google Scholar
Li H, Fan X, Houghton J. Tumor microenvironment: The role of the tumor stroma in cancer. J Cell Biochem. 2007;101:805–15.
CAS PubMed Google Scholar
Sahai E, Astsaturov I, Cukierman E, DeNardo DG, Egeblad M, Evans RM, et al. A framework for advancing our understanding of cancer-associated fibroblasts. Nat Rev Cancer. 2020;20:174–86.
CAS PubMed PubMed Central Google Scholar
Lavie D, Ben-Shmuel A, Erez N, Scherz-Shouval R. Cancer-associated fibroblasts in the single-cell era. Nat Cancer. 2022;3:793–807.
PubMed PubMed Central Google Scholar
Chen Y, McAndrews KM, Kalluri R. Clinical and therapeutic relevance of cancer-associated fibroblasts. Nat Rev Clin Oncol. 2021;18:792–804.
Biffi G, Tuveson DA. Diversity and Biology of Cancer-Associated Fibroblasts. Physiol Rev. 2021;101:147–76.
Kalluri R. The biology and function of fibroblasts in cancer. Nat Rev Cancer. 2016;16:582–98.
Mao X, Xu J, Wang W, Liang C, Hua J, Liu J, et al. Crosstalk between cancer-associated fibroblasts and immune cells in the tumor microenvironment: new findings and future perspectives. Mol Cancer. 2021;20:131.
Affo S, Nair A, Brundu F, Ravichandra A, Bhattacharjee S, Matsuda M, et al. Promotion of cholangiocarcinoma growth by diverse cancer-associated fibroblast subpopulations. Cancer Cell. 2021;39:866-882.e11.
Unterleuthner D, Neuhold P, Schwarz K, Janker L, Neuditschko B, Nivarthi H, et al. Cancer-associated fibroblast-derived WNT2 increases tumor angiogenesis in colon cancer. Angiogenesis. 2020;23:159–77.
Su S, Chen J, Yao H, Liu J, Yu S, Lao L, et al. CD10+GPR77+ Cancer-Associated Fibroblasts Promote Cancer Formation and Chemoresistance by Sustaining Cancer Stemness. Cell. 2018;172:841-856.e16.
Rhim AD, Oberstein PE, Thomas DH, Mirek ET, Palermo CF, Sastra SA, et al. Stromal Elements Act to Restrain, Rather Than Support. Pancreatic Ductal Adenocarcinoma Cancer Cell. 2014;25:735–47.
Bhattacharjee S, Hamberger F, Ravichandra A, Miller M, Nair A, Affo S, et al. Tumor restriction by type I collagen opposes tumor-promoting effects of cancer-associated fibroblasts. J Clin Investig. 2021;131: e146987.
Ahrends T, Borst J. The opposing roles of CD4+ T cells in anti-tumour immunity. Immunology. 2018;154:582–92.
van der Leun AM, Thommen DS, Schumacher TN. CD8+ T cell states in human cancer: insights from single-cell analysis. Nat Rev Cancer. 2020;20:218–32.
Li C, Jiang P, Wei S, Xu X, Wang J. Regulatory T cells in tumor microenvironment: new mechanisms, potential therapeutic strategies and future prospects. Mol Cancer. 2020;19:116.
Li Y-R, Wilson M, Yang L. Target tumor microenvironment by innate T cells. Front Immunol. 2022;13:999549.
Silva-Santos B, Mensurado S, Coffelt SB. γδ T cells: pleiotropic immune effectors with therapeutic potential in cancer. Nat Rev Cancer. 2019;19:392–404.
Downs-Canner SM, Meier J, Vincent BG, Serody JS. B Cell Function in the Tumor Microenvironment. Annu Rev Immunol. 2022;40:169–93.
Boutilier AJ, Elsawa SF. Macrophage Polarization States in the Tumor Microenvironment. Int J Mol Sci. 2021;22:6995.
Aponte-López A, Muñoz-Cruz S. Mast Cells in the Tumor Microenvironment. Adv Exp Med Biol. 2020;1273:159–73.
Guillerey C. NK Cells in the Tumor Microenvironment. Adv Exp Med Biol. 2020;1273:69–90.
Olingy CE, Dinh HQ, Hedrick CC. Monocyte heterogeneity and functions in cancer. J Leukoc Biol. 2019;106:309–22.
Hedrick CC, Malanchi I. Neutrophils in cancer: heterogeneous and multifaceted. Nat Rev Immunol. 2022;22:173–87.
Grisaru-Tal S, Itan M, Klion AD, Munitz A. A new dawn for eosinophils in the tumour microenvironment. Nat Rev Cancer. 2020;20:594–607.
Marone G, Gambardella AR, Mattei F, Mancini J, Schiavoni G, Varricchi G. Basophils in Tumor Microenvironment and Surroundings. Adv Exp Med Biol. 2020;1224:21–34.
Ridge SM, Sullivan FJ, Glynn SA. Mesenchymal stem cells: key players in cancer progression. Mol Cancer. 2017;16:31.
Li K, Shi H, Zhang B, Ou X, Ma Q, Chen Y, et al. Myeloid-derived suppressor cells as immunosuppressive regulators and therapeutic targets in cancer. Signal Transduct Target Ther. 2021;6:362.
Jiang Z, Zhou J, Li L, Liao S, He J, Zhou S, et al. Pericytes in the tumor microenvironment. Cancer Lett. 2023;556:216074.
Pallegar NK, Christian SL. Adipocytes in the Tumour Microenvironment. Adv Exp Med Biol. 2020;1234:1–13.
Jin M-Z, Jin W-L. The updated landscape of tumor microenvironment and drug repurposing. Signal Transduct Target Ther. 2020;5:166.
Bejarano L, Jordāo MJC, Joyce JA. Therapeutic Targeting of the Tumor Microenvironment. Cancer Discov. 2021;11:933–59.
de Visser KE, Joyce JA. The evolving tumor microenvironment: From cancer initiation to metastatic outgrowth. Cancer Cell. 2023;41:374–403.
St Paul M, Ohashi PS. The Roles of CD8+ T Cell Subsets in Antitumor Immunity. Trends Cell Biol. 2020;30:695–704.
Pan Y, Yu Y, Wang X, Zhang T. Tumor-Associated Macrophages in Tumor Immunity. Front Immunol. 2020;11:583084.
Wu K, Lin K, Li X, Yuan X, Xu P, Ni P, et al. Redefining Tumor-Associated Macrophage Subpopulations and Functions in the Tumor Microenvironment. Front Immunol. 2020;11:1731.
Verneau J, Sautés-Fridman C, Sun C-M. Dendritic cells in the tumor microenvironment: prognostic and theranostic impact. Semin Immunol. 2020;48:101410.
Garris CS, Luke JJ. Dendritic Cells, the T-cell-inflamed Tumor Microenvironment, and Immunotherapy Treatment Response. Clin Cancer Res. 2020;26:3901–7.
Komi DEA, Redegeld FA. Role of Mast Cells in Shaping the Tumor Microenvironment. Clin Rev Allergy Immunol. 2020;58:313–25.
Myers JA, Miller JS. Exploring the NK cell platform for cancer immunotherapy. Nat Rev Clin Oncol. 2021;18:85–100.
Ugel S, Canè S, De Sanctis F, Bronte V. Monocytes in the Tumor Microenvironment. Annu Rev Pathol. 2021;16:93–122.
Han X, Jorgensen JL, Brahmandam A, Schlette E, Huh YO, Shi Y, et al. Immunophenotypic study of basophils by multiparameter flow cytometry. Arch Pathol Lab Med. 2008;132:813–9.
Cromheecke JL, Nguyen KT, Huston DP. Emerging role of human basophil biology in health and disease. Curr Allergy Asthma Rep. 2014;14:408.
Zhang J, Yin H, Chen Q, Zhao G, Lou W, Wu W, et al. Basophils as a potential therapeutic target in cancer. J Zhejiang Univ Sci B. 2021;22:971–84.
Sağlam-Uçar Ö, Değirmenci İ, Halbutoğullari ZS, Pösteki G, Subaşi-Demirci C, Erman G, et al. The effect of mesenchymal stromal cells ın the microenvironment on cancer development. Med Oncol. 2022;39:114.
Whiteside TL. Exosome and mesenchymal stem cell cross-talk in the tumor microenvironment. Semin Immunol. 2018;35:69–79.
Wu Y, Yi M, Niu M, Mei Q, Wu K. Myeloid-derived suppressor cells: an emerging target for anticancer immunotherapy. Mol Cancer. 2022;21:184.
Li MO, Wolf N, Raulet DH, Akkari L, Pittet MJ, Rodriguez PC, et al. Innate immune cells in the tumor microenvironment. Cancer Cell. 2021;39:725–9.
Chen X. Turning foes to friends: targeting cancer-associated fibroblasts. DRug DiSCovERy. 2019;18(2):99–115.
Miyai Y, Esaki N, Takahashi M, Enomoto A. Cancer-associated fibroblasts that restrain cancer progression: Hypotheses and perspectives. Cancer Sci. 2020;111:1047–57.
Sun R, Kong X, Qiu X, Huang C, Wong P-P. The Emerging Roles of Pericytes in Modulating Tumor Microenvironment. Front Cell Dev Biol. 2021;9:676342.
Corn KC, Windham MA, Rafat M. Lipids in the tumor microenvironment: From cancer progression to treatment. Prog Lipid Res. 2020;80:101055.
Liu T, Han C, Wang S, Fang P, Ma Z, Xu L, et al. Cancer-associated fibroblasts: an emerging target of anti-cancer immunotherapy. J Hematol Oncol. 2019;12:86.
Muchlińska A, Nagel A, Popęda M, Szade J, Niemira M, Zieliński J, et al. Alpha-smooth muscle actin-positive cancer-associated fibroblasts secreting osteopontin promote growth of luminal breast cancer. Cell Mol Biol Lett. 2022;27:45.
Primac I, Maquoi E, Blacher S, Heljasvaara R, Van Deun J, Smeland HYH, et al. Stromal integrin α11 regulates PDGFRβ signaling and promotes breast cancer progression. J Clin Investig. 2019;129:4609–28.
Zeisberg EM, Potenta S, Xie L, Zeisberg M, Kalluri R. Discovery of Endothelial to Mesenchymal Transition as a Source for Carcinoma-Associated Fibroblasts. Can Res. 2007;67:10123–8.
CAS Google Scholar
Zhao L, Chen J, Pang Y, Fu K, Shang Q, Wu H, et al. Fibroblast activation protein-based theranostics in cancer research: A state-of-the-art review. Theranostics. 2022;12:1557–69.
Liu R, Li H, Liu L, Yu J, Ren X. Fibroblast activation protein: A potential therapeutic target in cancer. Cancer Biol Ther. 2012;13:123–9.
Huber MA, Schubert RD, Peter RU, Kraut N, Park JE, Rettig WJ, et al. Fibroblast Activation Protein: Differential Expression and Serine Protease Activity in Reactive Stromal Fibroblasts of Melanocytic Skin Tumors. J Investig Dermatol. 2003;120:182–8.
Nurmik M, Ullmann P, Rodriguez F, Haan S, Letellier E. In search of definitions: Cancer-associated fibroblasts and their markers. Int J Cancer. 2020;146:895–905.
Roy J, Hettiarachchi SU, Kaake M, Mukkamala R, Low PS. Design and validation of fibroblast activation protein alpha targeted imaging and therapeutic agents. Theranostics. 2020;10:5778–89.
Kraman M, Bambrough PJ, Arnold JN, Roberts EW, Magiera L, Jones JO, et al. Suppression of Antitumor Immunity by Stromal Cells Expressing Fibroblast Activation Protein–α. Science. 2010;330:827–30.
Li C, Teixeira AF, Zhu H-J, ten Dijke P. Cancer associated-fibroblast-derived exosomes in cancer progression. Mol Cancer. 2021;20:154.
Arina A, Idel C, Hyjek EM, Alegre M-L, Wang Y, Bindokas VP, et al. Tumor-associated fibroblasts predominantly come from local and not circulating precursors. Proc Natl Acad Sci USA. 2016;113:7551–6.
Yin C, Evason KJ, Asahina K, Stainier DYR. Hepatic stellate cells in liver development, regeneration, and cancer. J Clin Invest. 2013;123:1902–10.
Omary MB, Lugea A, Lowe AW, Pandol SJ. The pancreatic stellate cell: a star on the rise in pancreatic diseases. J Clin Invest. 2007;117:50–9.
Weber CE, Kothari AN, Wai PY, Li NY, Driver J, Zapf MAC, et al. Osteopontin mediates an MZF1–TGF-β1-dependent transformation of mesenchymal stem cells into cancer-associated fibroblasts in breast cancer. Oncogene. 2015;34:4821–33.
Jung Y, Kim JK, Shiozawa Y, Wang J, Mishra A, Joseph J, et al. Recruitment of mesenchymal stem cells into prostate tumours promotes metastasis. Nat Commun. 2013;4:1795.
Mishra PJ, Mishra PJ, Humeniuk R, Medina DJ, Alexe G, Mesirov JP, et al. Carcinoma-Associated Fibroblast-Like Differentiation of Human Mesenchymal Stem Cells. Can Res. 2008;68:4331–9.
Iwano M, Plieth D, Danoff TM, Xue C, Okada H, Neilson EG. Evidence that fibroblasts derive from epithelium during tissue fibrosis. J Clin Invest. 2002;110:341–50.
Sasaki K, Sugai T, Ishida K, Osakabe M, Amano H, Kimura H, et al. Analysis of cancer-associated fibroblasts and the epithelial-mesenchymal transition in cutaneous basal cell carcinoma, squamous cell carcinoma, and malignant melanoma. Hum Pathol. 2018;79:1–8.
Tang PC-T, Chung JY-F, Xiao J, Meng X-M, Huang X-R, et al. Smad3 Promotes Cancer-Associated Fibroblasts Generation via Macrophage-Myofibroblast Transition. Adv Sci (Weinh). 2022;9:e2101235.
Tang PM-K, Zhang Y-Y, Xiao J, Tang PC-T, Chung JY-F, Li J, et al. Neural transcription factor Pou4f1 promotes renal fibrosis via macrophage-myofibroblast transition. Proc Natl Acad Sci U S A. 2020;117:20741–52.
Hosaka K, Yang Y, Seki T, Fischer C, Dubey O, Fredlund E, et al. Pericyte–fibroblast transition promotes tumor growth and metastasis. Proc Natl Acad Sci USA. 2016;113. Available from: https://doi.org/10.1073/pnas.1608384113 . Cited 2022 Nov 17.
Bochet L, Lehuédé C, Dauvillier S, Wang YY, Dirat B, Laurent V, et al. Adipocyte-Derived Fibroblasts Promote Tumor Progression and Contribute to the Desmoplastic Reaction in Breast Cancer. Can Res. 2013;73:5657–68.
Lee K-W, Yeo S-Y, Gong J-R, Koo O-J, Sohn I, Lee WY, et al. PRRX1 is a master transcription factor of stromal fibroblasts for myofibroblastic lineage progression. Nat Commun. 2022;13:2793.
Kasashima H, Duran A, Martinez-Ordoñez A, Nakanishi Y, Kinoshita H, Linares JF, et al. Stromal SOX2 Upregulation Promotes Tumorigenesis through the Generation of a SFRP1/2-Expressing Cancer-Associated Fibroblast Population. Dev Cell. 2021;56:95-110.e10.
Halperin C, Hey J, Weichenhan D, Stein Y, Mayer S, Lutsik P, et al. Global DNA Methylation Analysis of Cancer-Associated Fibroblasts Reveals Extensive Epigenetic Rewiring Linked with RUNX1 Upregulation in Breast Cancer Stroma. Cancer Res. 2022;82:4139–52.
Li Q, Lv X, Han C, Kong Y, Dai Z, Huo D, et al. Enhancer reprogramming promotes the activation of cancer-associated fibroblasts and breast cancer metastasis. Theranostics. 2022;12:7491–508.
Kehrberg RJ, Bhyravbhatla N, Batra SK, Kumar S. Epigenetic regulation of cancer-associated fibroblast heterogeneity. Biochim Biophys Acta Rev Cancer. 2023;1878(3):188901.
Öhlund D, Handly-Santana A, Biffi G, Elyada E, Almeida AS, Ponz-Sarvise M, et al. Distinct populations of inflammatory fibroblasts and myofibroblasts in pancreatic cancer. J Exp Med. 2017;214:579–96.
Wang M, Feng R, Chen Z, Shi W, Li C, Liu H, et al. Identification of Cancer-Associated Fibroblast Subtype of Triple-Negative Breast Cancer. J Oncol. 2022;2022:6452636.
Bartoschek M, Oskolkov N, Bocci M, Lövrot J, Larsson C, Sommarin M, et al. Spatially and functionally distinct subclasses of breast cancer-associated fibroblasts revealed by single cell RNA sequencing. Nat Commun. 2018;9:5150.
Zhang L, Li Z, Skrzypczynska KM, Fang Q, Zhang W, O’Brien SA, et al. Single-Cell Analyses Inform Mechanisms of Myeloid-Targeted Therapies in Colon Cancer. Cell. 2020;181:442-459.e29.
Li H, Courtois ET, Sengupta D, Tan Y, Chen KH, Goh JJL, et al. Reference component analysis of single-cell transcriptomes elucidates cellular heterogeneity in human colorectal tumors. Nat Genet. 2017;49:708–18.
Ds F, M J, D D, Ke Y, M G, J G, et al. Multiomic analysis reveals conservation of cancer-associated fibroblast phenotypes across species and tissue of origin. Cancer cell [Internet]. 2022 [cited 2023 Mar 26];40. Available from: https://pubmed.ncbi.nlm.nih.gov/36270275/ .
Hu H, Piotrowska Z, Hare PJ, Chen H, Mulvey HE, Mayfield A, et al. Three subtypes of lung cancer fibroblasts define distinct therapeutic paradigms. Cancer Cell. 2021;39:1531-1547.e10.
Lambrechts D, Wauters E, Boeckx B, Aibar S, Nittner D, Burton O, et al. Phenotype molding of stromal cells in the lung tumor microenvironment. Nat Med. 2018;24:1277–89.
Pelon F, Bourachot B, Kieffer Y, Magagna I, Mermet-Meillon F, Bonnet I, et al. Cancer-associated fibroblast heterogeneity in axillary lymph nodes drives metastases in breast cancer through complementary mechanisms. Nat Commun. 2020;11:404.
Zhao Y, Mei S, Huang Y, Chen J, Zhang X, Zhang P. Integrative analysis deciphers the heterogeneity of cancer-associated fibroblast and implications on clinical outcomes in ovarian cancers. Comput Struct Biotechnol J. 2022;20:6403–11.
Galbo PM, Zang X, Zheng D. Molecular Features of Cancer-associated Fibroblast Subtypes and their Implication on Cancer Pathogenesis, Prognosis, and Immunotherapy Resistance. Clin Cancer Res. 2021;27:2636–47.
Elyada E, Bolisetty M, Laise P, Flynn WF, Courtois ET, Burkhart RA, et al. Cross-Species Single-Cell Analysis of Pancreatic Ductal Adenocarcinoma Reveals Antigen-Presenting Cancer-Associated Fibroblasts. Cancer Discov. 2019;9:1102–23.
Kieffer Y, Hocine HR, Gentric G, Pelon F, Bernard C, Bourachot B, et al. Single-Cell Analysis Reveals Fibroblast Clusters Linked to Immunotherapy Resistance in Cancer. Cancer Discov. 2020;10:1330–51.
Givel A-M, Kieffer Y, Scholer-Dahirel A, Sirven P, Cardon M, Pelon F, et al. miR200-regulated CXCL12β promotes fibroblast heterogeneity and immunosuppression in ovarian cancers. Nat Commun. 2018;9:1056.
Zheng S, Hu C, Lin H, Li G, Xia R, Zhang X, et al. circCUL2 induces an inflammatory CAF phenotype in pancreatic ductal adenocarcinoma via the activation of the MyD88-dependent NF-κB signaling pathway. J Exp Clin Cancer Res. 2022;41:71.
Li X, Sun Z, Peng G, Xiao Y, Guo J, Wu B, et al. Single-cell RNA sequencing reveals a pro-invasive cancer-associated fibroblast subgroup associated with poor clinical outcomes in patients with gastric cancer. Theranostics. 2022;12:620–38.
Dominguez CX, Müller S, Keerthivasan S, Koeppen H, Hung J, Gierke S, et al. Single-Cell RNA Sequencing Reveals Stromal Evolution into LRRC15+ Myofibroblasts as a Determinant of Patient Response to Cancer Immunotherapy. Cancer Discov. 2020;10:232–53.
Costa A, Kieffer Y, Scholer-Dahirel A, Pelon F, Bourachot B, Cardon M, et al. Fibroblast Heterogeneity and Immunosuppressive Environment in Human Breast Cancer. Cancer Cell. 2018;33:463-479.e10.
Houthuijzen JM, de Bruijn R, van der Burg E, Drenth AP, Wientjens E, Filipovic T, et al. CD26-negative and CD26-positive tissue-resident fibroblasts contribute to functionally distinct CAF subpopulations in breast cancer. Nat Commun. 2023;14:183.
Monteran L, Erez N. The Dark Side of Fibroblasts: Cancer-Associated Fibroblasts as Mediators of Immunosuppression in the Tumor Microenvironment. Front Immunol. 2019;10:1835.
Özdemir BC, Pentcheva-Hoang T, Carstens JL, Zheng X, Wu C-C, Simpson TR, et al. Depletion of Carcinoma-Associated Fibroblasts and Fibrosis Induces Immunosuppression and Accelerates Pancreas Cancer with Reduced Survival. Cancer Cell. 2014;25:719–34.
Chen Y, Kim J, Yang S, Wang H, Wu C-J, Sugimoto H, et al. Type I collagen deletion in αSMA+ myofibroblasts augments immune suppression and accelerates progression of pancreatic cancer. Cancer Cell. 2021;39:548-565.e6.
Hanahan D, Weinberg RA. Hallmarks of cancer: the next generation. Cell. 2011;144:646–74.
Li Z, Sun C, Qin Z. Metabolic reprogramming of cancer-associated fibroblasts and its effect on cancer cell reprogramming. Theranostics. 2021;11:8322–36.
Li Y, Zhao Z, Liu W, Li X. SNHG3 Functions as miRNA Sponge to Promote Breast Cancer Cells Growth Through the Metabolic Reprogramming. Appl Biochem Biotechnol. 2020;191:1084–99.
Kock A, Larsson K, Bergqvist F, Eissler N, Elfman LHM, Raouf J, et al. Inhibition of Microsomal Prostaglandin E Synthase-1 in Cancer-Associated Fibroblasts Suppresses Neuroblastoma Tumor Growth. EBioMedicine. 2018;32:84–92.
Elwakeel E, Brüggemann M, Wagih J, Lityagina O, Elewa MAF, Han Y, et al. Disruption of Prostaglandin E2 Signaling in Cancer-Associated Fibroblasts Limits Mammary Carcinoma Growth but Promotes Metastasis. Cancer Res. 2022;82:1380–95.
Peltier A, Seban R-D, Buvat I, Bidard F-C, Mechta-Grigoriou F. Fibroblast heterogeneity in solid tumors: From single cell analysis to whole-body imaging. Semin Cancer Biol. 2022;86:262–72.
Eckert MA, Coscia F, Chryplewicz A, Chang JW, Hernandez KM, Pan S, et al. Proteomics reveals NNMT as a master metabolic regulator of cancer-associated fibroblasts. Nature. 2019;569:723–8.
Wan X, Guan S, Hou Y, Qin Y, Zeng H, Yang L, et al. FOSL2 promotes VEGF-independent angiogenesis by transcriptionnally activating Wnt5a in breast cancer-associated fibroblasts. Theranostics. 2021;11:4975–91.
Apte RS, Chen DS, Ferrara N. VEGF in Signaling and Disease: Beyond Discovery and Development. Cell. 2019;176:1248–64.
De Francesco EM, Lappano R, Santolla MF, Marsico S, Caruso A, Maggiolini M. HIF-1α/GPER signaling mediates the expression of VEGF induced by hypoxia in breast cancer associated fibroblasts (CAFs). Breast Cancer Res. 2013;15:R64.
De Palma M, Biziato D, Petrova TV. Microenvironmental regulation of tumour angiogenesis. Nat Rev Cancer. 2017;17:457–74.
Tsai Y-M, Wu K-L, Liu Y-W, Chang W-A, Huang Y-C, Chang C-Y, et al. Cooperation Between Cancer and Fibroblasts in Vascular Mimicry and N2-Type Neutrophil Recruitment via Notch2-Jagged1 Interaction in Lung Cancer. Front Oncol. 2021;11:696931.
Yw S, Yd Z, Hz C, X L, Wd Z. Targeting CTGF in Cancer: An Emerging Therapeutic Opportunity. Trends in cancer. 2021;7. Available from: https://pubmed.ncbi.nlm.nih.gov/33358571/ . cited 2023 Feb 22.
J H, K V, C N, M R, Sb G, Tm J, et al. Activation of cancer-associated fibroblasts is required for tumor neovascularization in a murine model of melanoma. Matrix biology : journal of the International Society for Matrix Biology. 2018;74. Available from: https://pubmed.ncbi.nlm.nih.gov/29885461/ . Cited 2023 Feb 22.
Antonova DV, Zinovyeva MV, Kondratyeva LG, Sass AV, Alekseenko IV, Pleshkan VV. Possibility for Transcriptional Targeting of Cancer-Associated Fibroblasts-Limitations and Opportunities. Int J Mol Sci. 2021;22:3298.
Ohara Y, Enomoto A, Tsuyuki Y, Sato K, Iida T, Kobayashi H, et al. Connective tissue growth factor produced by cancer-associated fibroblasts correlates with poor prognosis in epithelioid malignant pleural mesothelioma. Oncol Rep. 2020;44:838–48.
Watanabe K, Shiga K, Maeda A, Harata S, Yanagita T, Suzuki T, et al. Chitinase 3-like 1 secreted from cancer-associated fibroblasts promotes tumor angiogenesis via interleukin-8 secretion in colorectal cancer. Int J Oncol. 2022;60:3.
Erdogan B, Ao M, White LM, Means AL, Brewer BM, Yang L, et al. Cancer-associated fibroblasts promote directional cancer cell migration by aligning fibronectin. J Cell Biol. 2017;216:3799–816.
Erdogan B, Webb DJ. Cancer-associated fibroblasts modulate growth factor signaling and extracellular matrix remodeling to regulate tumor metastasis. Biochem Soc Trans. 2017;45:229–36.
Hu D, Li Z, Zheng B, Lin X, Pan Y, Gong P, et al. Cancer-associated fibroblasts in breast cancer: Challenges and opportunities. Cancer Commun. 2022;42:401–34.
Google Scholar
Ishimoto T, Miyake K, Nandi T, Yashiro M, Onishi N, Huang KK, et al. Activation of Transforming Growth Factor Beta 1 Signaling in Gastric Cancer-associated Fibroblasts Increases Their Motility, via Expression of Rhomboid 5 Homolog 2, and Ability to Induce Invasiveness of Gastric Cancer Cells. Gastroenterology. 2017;153:191-204.e16.
Daniel SK, Seo YD, Pillarisetty VG. The CXCL12-CXCR4/CXCR7 axis as a mechanism of immune resistance in gastrointestinal malignancies. Semin Cancer Biol. 2020;65:176–88.
Izumi D, Ishimoto T, Miyake K, Sugihara H, Eto K, Sawayama H, et al. CXCL12/CXCR4 activation by cancer-associated fibroblasts promotes integrin β1 clustering and invasiveness in gastric cancer. Int J Cancer. 2016;138:1207–19.
Hemalatha SK, Sengodan SK, Nadhan R, Dev J, Sushama RR, Somasundaram V, et al. Brcal Defective Breast Cancer Cells Induce in vitro Transformation of Cancer Associated Fibroblasts (CAFs) to Metastasis Associated Fibroblasts (MAF). Sci Rep. 2018;8:13903.
Salimifard S, Masjedi A, Hojjat-Farsangi M, Ghalamfarsa G, Irandoust M, Azizi G, et al. Cancer associated fibroblasts as novel promising therapeutic targets in breast cancer. Pathol Res Pract. 2020;216:152915.
Sun X, He X, Zhang Y, Hosaka K, Andersson P, Wu J, et al. Inflammatory cell-derived CXCL3 promotes pancreatic cancer metastasis through a novel myofibroblast-hijacked cancer escape mechanism. Gut. 2022;71:129–47.
Vennin C, Mélénec P, Rouet R, Nobis M, Cazet AS, Murphy KJ, et al. CAF hierarchy driven by pancreatic cancer cell p53-status creates a pro-metastatic and chemoresistant environment via perlecan. Nat Commun. 2019;10:3637.
Elgundi Z, Papanicolaou M, Major G, Cox TR, Melrose J, Whitelock JM, et al. Cancer Metastasis: The Role of the Extracellular Matrix and the Heparan Sulfate Proteoglycan Perlecan. Front Oncol. 2019;9:1482.
Furini S, Falciani C. Expression and Role of Heparan Sulfated Proteoglycans in Pancreatic Cancer. Front Oncol. 2021;11:695858.
Zhou S-L, Dai Z, Zhou Z-J, Chen Q, Wang Z, Xiao Y-S, et al. CXCL5 contributes to tumor metastasis and recurrence of intrahepatic cholangiocarcinoma by recruiting infiltrative intratumoral neutrophils. Carcinogenesis. 2014;35:597–605.
Zhang W, Wang H, Sun M, Deng X, Wu X, Ma Y, et al. CXCL5/CXCR2 axis in tumor microenvironment as potential diagnostic biomarker and therapeutic target. Cancer Commun (Lond). 2020;40:69–80.
Xu H, Zhao J, Li J, Zhu Z, Cui Z, Liu R, et al. Cancer associated fibroblast-derived CCL5 promotes hepatocellular carcinoma metastasis through activating HIF1α/ZEB1 axis. Cell Death Dis. 2022;13:478.
Zhou Z, Cui D, Sun M-H, Huang J-L, Deng Z, Han B-M, et al. CAFs-derived MFAP5 promotes bladder cancer malignant behavior through NOTCH2/HEY1 signaling. FASEB J. 2020;34:7970–88.
Suh J, Kim D-H, Lee Y-H, Jang J-H, Surh Y-J. Fibroblast growth factor-2, derived from cancer-associated fibroblasts, stimulates growth and progression of human breast cancer cells via FGFR1 signaling. Mol Carcinog. 2020;59:1028–40.
Wen S, Hou Y, Fu L, Xi L, Yang D, Zhao M, et al. Cancer-associated fibroblast (CAF)-derived IL32 promotes breast cancer cell invasion and metastasis via integrin β3-p38 MAPK signalling. Cancer Lett. 2019;442:320–32.
Shani O, Vorobyov T, Monteran L, Lavie D, Cohen N, Raz Y, et al. Fibroblast-Derived IL33 Facilitates Breast Cancer Metastasis by Modifying the Immune Microenvironment and Driving Type 2 Immunity. Cancer Res. 2020;80:5317–29.
Paolillo S. Extracellular Matrix Alterations in Metastatic Processes. IJMS. 2019;20:4947.
Tr C. The matrix in cancer. Nature reviews Cancer. 2021 ;21. Available from: https://pubmed.ncbi.nlm.nih.gov/33589810/ . Cited 2023 Jan 2.
Walker C, Mojares E, Del Río HA. Role of Extracellular Matrix in Development and Cancer Progression. Int J Mol Sci. 2018;19:3028.
Xiao Q, Ge G. Lysyl Oxidase, Extracellular Matrix Remodeling and Cancer Metastasis. Cancer Microenvironment. 2012;5:261–73.
Zhang Y, Gu Z, Wan J, Lou X, Liu S, Wang Y, et al. Stearoyl-CoA Desaturase-1 dependent lipid droplets accumulation in cancer-associated fibroblasts facilitates the progression of lung cancer. Int J Biol Sci. 2022;18:6114–28.
Min K-W, Kim D-H, Noh Y-K, Son BK, Kwon MJ, Moon J-Y. Cancer-associated fibroblasts are associated with poor prognosis in solid type of lung adenocarcinoma in a machine learning analysis. Sci Rep. 2021;11:16779.
Nguyen EV, Pereira BA, Lawrence MG, Ma X, Rebello RJ, Chan H, et al. Proteomic Profiling of Human Prostate Cancer-associated Fibroblasts (CAF) Reveals LOXL2-dependent Regulation of the Tumor Microenvironment. Mol Cell Proteomics. 2019;18:1410–27.
Wei L, Ye H, Li G, Lu Y, Zhou Q, Zheng S, et al. Cancer-associated fibroblasts promote progression and gemcitabine resistance via the SDF-1/SATB-1 pathway in pancreatic cancer. Cell Death Dis. 2018;9:1065.
Cheteh EH, Sarne V, Ceder S, Bianchi J, Augsten M, Rundqvist H, et al. Interleukin-6 derived from cancer-associated fibroblasts attenuates the p53 response to doxorubicin in prostate cancer cells. Cell Death Discov. 2020;6:42.
Whibley C, Pharoah PDP, Hollstein M. p53 polymorphisms: cancer implications. Nat Rev Cancer. 2009;9:95–107.
Gao Y, Li X, Zeng C, Liu C, Hao Q, Li W, et al. CD63+ Cancer-Associated Fibroblasts Confer Tamoxifen Resistance to Breast Cancer Cells through Exosomal miR-22. Adv Sci (Weinh). 2020;7:2002518.
Yan L, Wang P, Fang W, Liang C. Cancer-associated fibroblasts-derived exosomes-mediated transfer of LINC00355 regulates bladder cancer cell proliferation and invasion. Cell Biochem Funct. 2020;38:257–65.
Luo G, Zhang Y, Wu Z, Zhang L, Liang C, Chen X. Exosomal LINC00355 derived from cancer-associated fibroblasts promotes bladder cancer cell resistance to cisplatin by regulating miR-34b-5p/ABCB1 axis. Acta Biochim Biophys Sin (Shanghai). 2021;53:558–66.
Zhang Y, Luo G, You S, Zhang L, Liang C, Chen X. Exosomal LINC00355 derived from cancer-associated fibroblasts promotes bladder cancer cell proliferation and invasion by regulating miR-15a-5p/HMGA2 axis. Acta Biochim Biophys Sin (Shanghai). 2021;53:673–82.
Fang Y, Zhou W, Rong Y, Kuang T, Xu X, Wu W, et al. Exosomal miRNA-106b from cancer-associated fibroblast promotes gemcitabine resistance in pancreatic cancer. Exp Cell Res. 2019;383:111543.
Haldar S, Mishra R, Billet S, Thiruvalluvan M, Placencio-Hickok VR, Madhav A, et al. Cancer epithelia-derived mitochondrial DNA is a targetable initiator of a paracrine signaling loop that confers taxane resistance. Proc Natl Acad Sci U S A. 2020;117:8515–23.
Liu Y, Xun Z, Ma K, Liang S, Li X, Zhou S, et al. Identification of a tumour immune barrier in the HCC microenvironment that determines the efficacy of immunotherapy. J Hepatol. 2023;78:770–82.
Liu L, Liu S, Luo H, Chen C, Zhang X, He L, et al. GPR30-mediated HMGB1 upregulation in CAFs induces autophagy and tamoxifen resistance in ERα-positive breast cancer cells. Aging (Albany NY). 2021;13:16178–97.
Ni Y, Zhou X, Yang J, Shi H, Li H, Zhao X, et al. The Role of Tumor-Stroma Interactions in Drug Resistance Within Tumor Microenvironment. Front Cell Dev Biol. 2021;9:637675.
Zhao C, Liu S, Gao F, Zou Y, Ren Z, Yu Z. The role of tumor microenvironment reprogramming in primary liver cancer chemotherapy resistance. Front Oncol. 2022;12:1008902.
Zhang H, Deng T, Liu R, Ning T, Yang H, Liu D, et al. CAF secreted miR-522 suppresses ferroptosis and promotes acquired chemo-resistance in gastric cancer. Mol Cancer. 2020;19:43.
Zhao J, Yang S, Lv C, Liu Y. Cancer associated fibroblasts suppressed ferroptosis in glioblastoma via upregulating lncRNA DLEU1. Am J Physiol Cell Physiol. 2023;324(5):C1039–52.
Erkan M, Hausmann S, Michalski CW, Fingerle AA, Dobritz M, Kleeff J, et al. The role of stroma in pancreatic cancer: diagnostic and therapeutic implications. Nat Rev Gastroenterol Hepatol. 2012;9:454–67.
Hosein AN, Brekken RA, Maitra A. Pancreatic cancer stroma: an update on therapeutic targeting strategies. Nat Rev Gastroenterol Hepatol. 2020;17:487–505.
Flavell RA, Sanjabi S, Wrzesinski SH, Licona-Limón P. The polarization of immune cells in the tumour environment by TGFbeta. Nat Rev Immunol. 2010;10:554–67.
Teicher BA, Fricker SP. CXCL12 (SDF-1)/CXCR4 pathway in cancer. Clin Cancer Res. 2010;16:2927–31.
Santagata S, Ieranò C, Trotta AM, Capiluongo A, Auletta F, Guardascione G, et al. CXCR4 and CXCR7 Signaling Pathways: A Focus on the Cross-Talk Between Cancer Cells and Tumor Microenvironment. Front Oncol. 2021;11:591386.
Wei R, Zhou Y, Li C, Rychahou P, Zhang S, Titlow WB, et al. Ketogenesis Attenuates KLF5-Dependent Production of CXCL12 to Overcome the Immunosuppressive Tumor Microenvironment in Colorectal Cancer. Cancer Res. 2022;82:1575–88.
Wu X, Qian L, Zhao H, Lei W, Liu Y, Xu X, et al. CXCL12/CXCR4: An amazing challenge and opportunity in the fight against fibrosis. Ageing Res Rev. 2023;83:101809.
Peng D, Fu M, Wang M, Wei Y, Wei X. Targeting TGF-β signal transduction for fibrosis and cancer therapy. Mol Cancer. 2022;21:104.
Ringuette Goulet C, Bernard G, Tremblay S, Chabaud S, Bolduc S, Pouliot F. Exosomes Induce Fibroblast Differentiation into Cancer-Associated Fibroblasts through TGFβ Signaling. Mol Cancer Res. 2018;16:1196–204.
Wu F, Yang J, Liu J, Wang Y, Mu J, Zeng Q, et al. Signaling pathways in cancer-associated fibroblasts and targeted therapy for cancer. Signal Transduct Target Ther. 2021;6:218.
Huang M, Fu M, Wang J, Xia C, Zhang H, Xiong Y, et al. TGF-β1-activated cancer-associated fibroblasts promote breast cancer invasion, metastasis and epithelial-mesenchymal transition by autophagy or overexpression of FAP-α. Biochem Pharmacol. 2021;188:114527.
Ham I-H, Oh HJ, Jin H, Bae CA, Jeon S-M, Choi KS, et al. Targeting interleukin-6 as a strategy to overcome stroma-induced resistance to chemotherapy in gastric cancer. Mol Cancer. 2019;18:68.
Xiang X, Niu Y-R, Wang Z-H, Ye L-L, Peng W-B, Zhou Q. Cancer-associated fibroblasts: Vital suppressors of the immune response in the tumor microenvironment. Cytokine Growth Factor Rev. 2022;67:35–48.
Y D, J C, B F, W L, G C, Q Z, et al. Hepatic carcinoma-associated fibroblasts enhance immune suppression by facilitating the generation of myeloid-derived suppressor cells. Oncogene. 2017;36. Available from: https://pubmed.ncbi.nlm.nih.gov/27593937/ . Cited 2023 Jan 18.
Avalle L, Raggi L, Monteleone E, Savino A, Viavattene D, Statello L, et al. STAT3 induces breast cancer growth via ANGPTL4, MMP13 and STC1 secretion by cancer associated fibroblasts. Oncogene. 2022;41:1456–67.
Zou S, Tong Q, Liu B, Huang W, Tian Y, Fu X. Targeting STAT3 in Cancer Immunotherapy. Mol Cancer. 2020;19:145.
Yang X, Lin Y, Shi Y, Li B, Liu W, Yin W, et al. FAP Promotes Immunosuppression by Cancer-Associated Fibroblasts in the Tumor Microenvironment via STAT3–CCL2 Signaling. Can Res. 2016;76:4124–35.
Kato T, Noma K, Ohara T, Kashima H, Katsura Y, Sato H, et al. Cancer-Associated Fibroblasts Affect Intratumoral CD8+ and FoxP3+ T Cells Via IL6 in the Tumor Microenvironment. Clin Cancer Res. 2018;24:4820–33.
Farhood B, Najafi M, Mortezaee K. CD8+ cytotoxic T lymphocytes in cancer immunotherapy: A review. J Cell Physiol. 2019;234:8509–21.
Jenkins L, Jungwirth U, Avgustinova A, Iravani M, Mills A, Haider S, et al. Cancer-Associated Fibroblasts Suppress CD8+ T-cell Infiltration and Confer Resistance to Immune-Checkpoint Blockade. Cancer Res. 2022;82:2904–17.
Lakins MA, Ghorani E, Munir H, Martins CP, Shields JD. Cancer-associated fibroblasts induce antigen-specific deletion of CD8 + T Cells to protect tumour cells. Nat Commun. 2018;9:948.
Murai H, Kodama T, Maesaka K, Tange S, Motooka D, Suzuki Y, et al. Multiomics identifies the link between intratumor steatosis and the exhausted tumor immune microenvironment in hepatocellular carcinoma. Hepatology. 2023;77:77–91.
Huang H, Wang Z, Zhang Y, Pradhan RN, Ganguly D, Chandra R, et al. Mesothelial cell-derived antigen-presenting cancer-associated fibroblasts induce expansion of regulatory T cells in pancreatic cancer. Cancer Cell. 2022;40:656-673.e7.
Burley A, Rullan A, Wilkins A. A review of the biology and therapeutic implications of cancer-associated fibroblasts (CAFs) in muscle-invasive bladder cancer. Front Oncol. 2022;12:1000888.
Suzuki J, Aokage K, Neri S, Sakai T, Hashimoto H, Su Y, et al. Relationship between podoplanin-expressing cancer-associated fibroblasts and the immune microenvironment of early lung squamous cell carcinoma. Lung Cancer. 2021;153:1–10.
Gil-Julio H, Perea F, Rodriguez-Nicolas A, Cozar JM, González-Ramirez AR, Concha A, et al. Tumor Escape Phenotype in Bladder Cancer Is Associated with Loss of HLA Class I Expression, T-Cell Exclusion and Stromal Changes. Int J Mol Sci. 2021;22:7248.
Mhaidly R, Mechta-Grigoriou F. Role of cancer-associated fibroblast subpopulations in immune infiltration, as a new means of treatment in cancer. Immunol Rev. 2021;302:259–72.
Sun C, Mezzadra R, Schumacher TN. Regulation and Function of the PD-L1 Checkpoint. Immunity. 2018;48:434–52.
Dou D, Ren X, Han M, Xu X, Ge X, Gu Y, et al. Cancer-Associated Fibroblasts-Derived Exosomes Suppress Immune Cell Function in Breast Cancer via the miR-92/PD-L1 Pathway. Front Immunol. 2020;11:2026.
Grout JA, Sirven P, Leader AM, Maskey S, Hector E, Puisieux I, et al. Spatial Positioning and Matrix Programs of Cancer-Associated Fibroblasts Promote T-cell Exclusion in Human Lung Tumors. Cancer Discov. 2022;12:2606–25.
Sazeides C, Le A. Metabolic Relationship Between Cancer-Associated Fibroblasts and Cancer Cells. Adv Exp Med Biol. 2021;1311:189–204.
Li J, Qi D, Hsieh T-C, Huang JH, Wu JM, Wu E. Trailblazing perspectives on targeting breast cancer stem cells. Pharmacol Ther. 2021;223:107800.
Nallasamy P, Nimmakayala RK, Karmakar S, Leon F, Seshacharyulu P, Lakshmanan I, et al. Pancreatic Tumor Microenvironment Factor Promotes Cancer Stemness via SPP1-CD44 Axis. Gastroenterology. 2021;161:1998-2013.e7.
Fujii N, Yashiro M, Hatano T, Fujikawa H, Motomura H. CD9-positive Exosomes Derived from Cancer-associated Fibroblasts Might Inhibit the Proliferation of Malignant Melanoma Cells. Anticancer Res. 2023;43:25–33.
Ishibashi M, Neri S, Hashimoto H, Miyashita T, Yoshida T, Nakamura Y, et al. CD200-positive cancer associated fibroblasts augment the sensitivity of Epidermal Growth Factor Receptor mutation-positive lung adenocarcinomas to EGFR Tyrosine kinase inhibitors. Sci Rep. 2017;7:46662.
Remsing Rix LL, Sumi NJ, Hu Q, Desai B, Bryant AT, Li X, et al. IGF-binding proteins secreted by cancer-associated fibroblasts induce context-dependent drug sensitization of lung cancer cells. Sci Signal. 2022;15(eabj5879).
Lee JJ, Perera RM, Wang H, Wu D-C, Liu XS, Han S, et al. Stromal response to Hedgehog signaling restrains pancreatic cancer progression. Proc Natl Acad Sci U S A. 2014;111:E3091-3100.
Park D, Sahai E. Rullan A. SnapShot: Cancer-Associated Fibroblasts. Cell. 2020;181:486-486.e1.
Tanaka R, Kimura K, Eguchi S, Ohira G, Tanaka S, Amano R, et al. Interleukin-8 produced from cancer-associated fibroblasts suppresses proliferation of the OCUCh-LM1 cancer cell line. BMC Cancer. 2022;22:748.
Yamada N, Chung YS, Arimoto Y, Sawada T, Seki S, Sowa M. Establishment of a new human extrahepatic bile duct carcinoma cell line (OCUCh-LM1) and experimental liver metastatic model. Br J Cancer. 1995;71:543–8.
Wienke D, Davies GC, Johnson DA, Sturge J, Lambros MBK, Savage K, et al. The collagen receptor Endo180 (CD280) Is expressed on basal-like breast tumor cells and promotes tumor growth in vivo. Cancer Res. 2007;67:10230–40.
Jungwirth U, van Weverwijk A, Evans RJ, Jenkins L, Vicente D, Alexander J, et al. Impairment of a distinct cancer-associated fibroblast population limits tumour growth and metastasis. Nat Commun. 2021;12:3516.
Pan K, Farrukh H, Chittepu VCSR, Xu H, Pan C-X, Zhu Z. CAR race to cancer immunotherapy: from CAR T, CAR NK to CAR macrophage therapy. J Exp Clin Cancer Res. 2022;41:119.
Younes A, Berdeja JG, Patel MR, Flinn I, Gerecitano JF, Neelapu SS, et al. Safety, tolerability, and preliminary activity of CUDC-907, a first-in-class, oral, dual inhibitor of HDAC and PI3K, in patients with relapsed or refractory lymphoma or multiple myeloma: an open-label, dose-escalation, phase 1 trial. Lancet Oncol. 2016;17:622–31.
Yeh C-R, Slavin S, Da J, Hsu I, Luo J, Xiao G-Q, et al. Estrogen receptor α in cancer associated fibroblasts suppresses prostate cancer invasion via reducing CCL5, IL6 and macrophage infiltration in the tumor microenvironment. Mol Cancer. 2016;15:7.
Slavin S, Yeh C-R, Da J, Yu S, Miyamoto H, Messing EM, et al. Estrogen receptor α in cancer-associated fibroblasts suppresses prostate cancer invasion via modulation of thrombospondin 2 and matrix metalloproteinase 3. Carcinogenesis. 2014;35:1301–9.
Britt KL, Cuzick J, Phillips K-A. Key steps for effective breast cancer prevention. Nat Rev Cancer. 2020;20:417–36.
Fox S, Speirs V, Shaaban AM. Male breast cancer: an update. Virchows Arch. 2022;480:85–93.
Paluch-Shimon S, Cardoso F, Partridge AH, Abulkhair O, Azim HA, Bianchi-Micheli G, et al. ESO-ESMO fifth international consensus guidelines for breast cancer in young women (BCY5). Ann Oncol. 2022;33:1097–118.
Trapani D, Ginsburg O, Fadelu T, Lin NU, Hassett M, Ilbawi AM, et al. Global challenges and policy solutions in breast cancer control. Cancer Treat Rev. 2022;104:102339.
Harbeck N, Gnant M. Breast cancer. Lancet. 2017;389:1134–50.
Ostermann E, Garin-Chesa P, Heider KH, Kalat M, Lamche H, Puri C, et al. Effective Immunoconjugate Therapy in Cancer Models Targeting a Serine Protease of Tumor Fibroblasts. Clin Cancer Res. 2008;14:4584–92.
Fang J, Xiao L, Joo K-I, Liu Y, Zhang C, Liu S, et al. A potent immunotoxin targeting fibroblast activation protein for treatment of breast cancer in mice: Immunotoxin targeting fibroblast activation protein. Int J Cancer. 2016;138:1013–23.
Kakarla S, Song X-T, Gottschalk S. Cancer-associated fibroblasts as targets for immunotherapy. Immunotherapy. 2012;4:1129–38.
Loeffler M. Targeting tumor-associated fibroblasts improves cancer chemotherapy by increasing intratumoral drug uptake. J Clin Investig. 2006;116:1955–62.
Duperret EK, Trautz A, Ammons D, Perales-Puchalt A, Wise MC, Yan J, et al. Alteration of the Tumor Stroma Using a Consensus DNA Vaccine Targeting Fibroblast Activation Protein (FAP) Synergizes with Antitumor Vaccine Therapy in Mice. Clin Cancer Res. 2018;24:1190–201.
Zhang F-F, Qiao Y, Xie Y, Liu C, Wu H, Wu J-X, et al. Epitope-based minigene vaccine targeting fibroblast activation protein α induces specific immune responses and anti-tumor effects in 4 T1 murine breast cancer model. Int Immunopharmacol. 2022;112:109237.
Hofheinz R-D, al-Batran S-E, Hartmann F, Hartung G, Jäger D, Renner C, et al. Stromal Antigen Targeting by a Humanised Monoclonal Antibody: An Early Phase II Trial of Sibrotuzumab in Patients with Metastatic Colorectal Cancer. Oncol Res Treat. 2003;26:44–8.
Narra K, Mullins SR, Lee H-O, Strzemkowski-Brun B, Magalong K, Christiansen VJ, et al. Phase II trial of single agent Val-boroPro (talabostat) inhibiting fibroblast activation protein in patients with metastatic colorectal cancer. Cancer Biol Ther. 2007;6:1691–9.
Elboga U, Sahin E, Kus T, Cayirli YB, Aktas G, Uzun E, et al. Superiority of 68Ga-FAPI PET/CT scan in detecting additional lesions compared to 18FDG PET/CT scan in breast cancer. Ann Nucl Med. 2021;35:1321–31.
Zboralski D, Hoehne A, Bredenbeck A, Schumann A, Nguyen M, Schneider E, et al. Preclinical evaluation of FAP-2286 for fibroblast activation protein targeted radionuclide imaging and therapy. Eur J Nucl Med Mol Imaging. 2022;49:3651–67.
Dendl K, Koerber SA, Finck R, Mokoala KMG, Staudinger F, Schillings L, et al. 68Ga-FAPI-PET/CT in patients with various gynecological malignancies. Eur J Nucl Med Mol Imaging. 2021;48:4089–100.
Ariga N, Sato E, Ohuchi N, Nagura H, Ohtani H. Stromal expression of fibroblast activation protein/seprase, a cell membrane serine proteinase and gelatinase, is associated with longer survival in patients with invasive ductal carcinoma of breast. Int J Cancer. 2001;95:67–72.
Tran E, Chinnasamy D, Yu Z, Morgan RA, Lee C-CR, Restifo NP, et al. Immune targeting of fibroblast activation protein triggers recognition of multipotent bone marrow stromal cells and cachexia. J Exp Med. 2013;210:1125–35.
Burstein HJ. The distinctive nature of HER2-positive breast cancers. N Engl J Med. 2005;353:1652–4.
Fernández-Nogueira P, Fuster G, Gutierrez-Uzquiza Á, Gascón P, Carbó N, Bragado P. Cancer-Associated Fibroblasts in Breast Cancer Treatment Response and Metastasis. Cancers (Basel). 2021;13:3146.
Rivas EI, Linares J, Zwick M, Gómez-Llonin A, Guiu M, Labernadie A, et al. Targeted immunotherapy against distinct cancer-associated fibroblasts overcomes treatment resistance in refractory HER2+ breast tumors. Nat Commun. 2022;13:5310.
Waldhauer I, Gonzalez-Nicolini V, Freimoser-Grundschober A, Nayak TK, Fahrni L, Hosse RJ, et al. Simlukafusp alfa (FAP-IL2v) immunocytokine is a versatile combination partner for cancer immunotherapy. MAbs. 2021;13:1913791.
Liu S, Ren J, Ten Dijke P. Targeting TGFβ signal transduction for cancer therapy. Signal Transduct Target Ther. 2021;6:8.
Kojima Y, Acar A, Eaton EN, Mellody KT, Scheel C, Ben-Porath I, et al. Autocrine TGF-beta and stromal cell-derived factor-1 (SDF-1) signaling drives the evolution of tumor-promoting mammary stromal myofibroblasts. Proc Natl Acad Sci U S A. 2010;107:20009–14.
Tang X, Tu G, Yang G, Wang X, Kang L, Yang L, et al. Autocrine TGF-β1/miR-200s/miR-221/DNMT3B regulatory loop maintains CAF status to fuel breast cancer cell proliferation. Cancer Lett. 2019;452:79–89.
Yu Y, Xiao C-H, Tan L-D, Wang Q-S, Li X-Q, Feng Y-M. Cancer-associated fibroblasts induce epithelial-mesenchymal transition of breast cancer cells through paracrine TGF-β signalling. Br J Cancer. 2014;110:724–32.
Ren Y, Jia H-H, Xu Y-Q, Zhou X, Zhao X-H, Wang Y-F, et al. Paracrine and epigenetic control of CAF-induced metastasis: the role of HOTAIR stimulated by TGF-ß1 secretion. Mol Cancer. 2018;17:5.
Li Q, Yuan H, Zhao G, Zhang J, Li S, Gong D, et al. ZNF32 prevents the activation of cancer-associated fibroblasts through negative regulation of TGFB1 transcription in breast cancer. FASEB J. 2023;37:e22837.
Formenti SC, Lee P, Adams S, Goldberg JD, Li X, Xie MW, et al. Focal Irradiation and Systemic TGFβ Blockade in Metastatic Breast Cancer. Clin Cancer Res. 2018;24:2493–504.
den Hollander MW, Bensch F, Glaudemans AWJM, Oude Munnink TH, Enting RH, den Dunnen WFA, et al. TGF-β Antibody Uptake in Recurrent High-Grade Glioma Imaged with 89Zr-Fresolimumab PET. J Nucl Med. 2015;56:1310–4.
Lan Y, Yeung T-L, Huang H, Wegener AA, Saha S, Toister-Achituv M, et al. Colocalized targeting of TGF-β and PD-L1 by bintrafusp alfa elicits distinct antitumor responses. J Immunother Cancer. 2022;10:e004122.
Tschernia NP, Gulley JL. Tumor in the Crossfire: Inhibiting TGF-β to Enhance Cancer Immunotherapy. BioDrugs. 2022;36:153–80.
Chandra Jena B, Kanta Das C, Banerjee I, Das S, Bharadwaj D, Majumder R, et al. Paracrine TGF-β1 from breast cancer contributes to chemoresistance in cancer associated fibroblasts via upregulation of the p44/42 MAPK signaling pathway. Biochem Pharmacol. 2021;186:114474.
Chauhan VP, Chen IX, Tong R, Ng MR, Martin JD, Naxerova K, et al. Reprogramming the microenvironment with tumor-selective angiotensin blockers enhances cancer immunotherapy. Proc Natl Acad Sci U S A. 2019;116:10674–80.
Hauge A, Rofstad EK. Antifibrotic therapy to normalize the tumor microenvironment. J Transl Med. 2020;18:207.
Zhao Q, He X, Qin X, Liu Y, Jiang H, Wang J, et al. Enhanced Therapeutic Efficacy of Combining Losartan and Chemo-Immunotherapy for Triple Negative Breast Cancer. Front Immunol. 2022;13:938439.
Sung B, Jhurani S, Ahn KS, Mastuo Y, Yi T, Guha S, et al. Zerumbone down-regulates chemokine receptor CXCR4 expression leading to inhibition of CXCL12-induced invasion of breast and pancreatic tumor cells. Cancer Res. 2008;68:8938–44.
Al-Amin M, Eltayeb NM, Hossain CF, Khairuddean M, Fazalul Rahiman SS, Salhimi SM. Inhibitory Activity of Extract, Fractions, and Compounds from Zingiber montanum Rhizomes on the Migration of Breast Cancer Cells. Planta Med. 2020;86:387–94.
Kim S, Lee J, Jeon M, Lee JE, Nam SJ. Zerumbone suppresses the motility and tumorigenecity of triple negative breast cancer cells via the inhibition of TGF-β1 signaling pathway. Oncotarget. 2016;7:1544–58.
Li J, Wang L, Sun Y, Wang Z, Qian Y, Duraisamy V, et al. Zerumbone-induced reactive oxygen species-mediated oxidative stress re-sensitizes breast cancer cells to paclitaxel. Biotechnol Appl Biochem. 2022;70(1):28–37.
Xu C, Zhao H, Chen H, Yao Q. CXCR4 in breast cancer: oncogenic role and therapeutic targeting. Drug Des Devel Ther. 2015;9:4953–64.
Martinez-Ordoñez A, Seoane S, Cabezas P, Eiro N, Sendon-Lago J, Macia M, et al. Breast cancer metastasis to liver and lung is facilitated by Pit-1-CXCL12-CXCR4 axis. Oncogene. 2018;37:1430–44.
Hinton CV, Avraham S, Avraham HK. Role of the CXCR4/CXCL12 signaling axis in breast cancer metastasis to the brain. Clin Exp Metastasis. 2010;27:97–105.
Zielińska KA, Katanaev VL. The Signaling Duo CXCL12 and CXCR4: Chemokine Fuel for Breast Cancer Tumorigenesis. Cancers (Basel). 2020;12:3071.
Orimo A, Gupta PB, Sgroi DC, Arenzana-Seisdedos F, Delaunay T, Naeem R, et al. Stromal fibroblasts present in invasive human breast carcinomas promote tumor growth and angiogenesis through elevated SDF-1/CXCL12 secretion. Cell. 2005;121:335–48.
Timperi E, Gueguen P, Molgora M, Magagna I, Kieffer Y, Lopez-Lastra S, et al. Lipid-Associated Macrophages Are Induced by Cancer-Associated Fibroblasts and Mediate Immune Suppression in Breast Cancer. Cancer Res. 2022;82:3291–306.
Chen IX, Chauhan VP, Posada J, Ng MR, Wu MW, Adstamongkonkul P, et al. Blocking CXCR4 alleviates desmoplasia, increases T-lymphocyte infiltration, and improves immunotherapy in metastatic breast cancer. Proc Natl Acad Sci U S A. 2019;116:4558–66.
Pan H, Peng Z, Lin J, Ren X, Zhang G, Cui Y. Forkhead box C1 boosts triple-negative breast cancer metastasis through activating the transcription of chemokine receptor-4. Cancer Sci. 2018;109:3794–804.
Zhou J, Le K, Xu M, Ming J, Yang W, Zhang Q, et al. CXCR4 Antagonist AMD3100 Reverses the Resistance to Tamoxifen in Breast Cancer via Inhibiting AKT Phosphorylation. Mol Ther Oncolytics. 2020;18:161–70.
Smith MCP, Luker KE, Garbow JR, Prior JL, Jackson E, Piwnica-Worms D, et al. CXCR4 regulates growth of both primary and metastatic breast cancer. Cancer Res. 2004;64:8604–12.
Biasci D, Smoragiewicz M, Connell CM, Wang Z, Gao Y, Thaventhiran JED, et al. CXCR4 inhibition in human pancreatic and colorectal cancers induces an integrated immune response. Proc Natl Acad Sci U S A. 2020;117:28960–70.
Chen Y, Ramjiawan RR, Reiberger T, Ng MR, Hato T, Huang Y, et al. CXCR4 inhibition in tumor microenvironment facilitates anti-programmed death receptor-1 immunotherapy in sorafenib-treated hepatocellular carcinoma in mice. Hepatology. 2015;61:1591–602.
Harper MM, Lin M, Cavnar MJ, Pandalai PK, Patel RA, Gao M, et al. Interaction of immune checkpoint PD-1 and chemokine receptor 4 (CXCR4) promotes a malignant phenotype in pancreatic cancer cells. PLoS ONE. 2022;17:e0270832.
Wu Y, Yi Z, Li J, Wei Y, Feng R, Liu J, et al. FGFR blockade boosts T cell infiltration into triple-negative breast cancer by regulating cancer-associated fibroblasts. Theranostics. 2022;12:4564–80.
Singh S, Lamichhane A, Rafsanjani Nejad P, Heiss J, Baumann H, Gudneppanavar R, et al. Therapeutic Targeting of Stromal-Tumor HGF-MET Signaling in an Organotypic Triple-Negative Breast Tumor Model. Mol Cancer Res. 2022;20:1166–77.
Wang B, Liu W, Liu C, Du K, Guo Z, Zhang G, et al. Cancer-Associated Fibroblasts Promote Radioresistance of Breast Cancer Cells via the HGF/c-Met Signaling Pathway. Int J Radiat Oncol Biol Phys. 2022;S0360–3016(22):03679–83.
Yi Y, Zeng S, Wang Z, Wu M, Ma Y, Ye X, et al. Cancer-associated fibroblasts promote epithelial-mesenchymal transition and EGFR-TKI resistance of non-small cell lung cancers via HGF/IGF-1/ANXA2 signaling. Biochim Biophys Acta Mol Basis Dis. 2018;1864:793–803.
Qin H, Yang Y, Jiang B, Pan C, Chen W, Diao W, et al. SOX9 in prostate cancer is upregulated by cancer-associated fibroblasts to promote tumor progression through HGF/c-Met-FRA1 signaling. FEBS J. 2021;288:5406–29.
Guo Z, Zhang H, Fu Y, Kuang J, Zhao B, Zhang L, et al. Cancer-associated fibroblasts induce growth and radioresistance of breast cancer cells through paracrine IL-6. Cell Death Discov. 2023;9:6.
Dittmer A, Dittmer J. A CAF-Fueled TIMP-1/CD63/ITGB1/STAT3 Feedback Loop Promotes Migration and Growth of Breast Cancer Cells. Cancers (Basel). 2022;14:4983.
Ji P, Xu X, Ma S, Fan J, Zhou Q, Mao X, et al. Novel 2-Carbonylbenzo[b]thiophene 1,1-Dioxide Derivatives as Potent Inhibitors of STAT3 Signaling Pathway. ACS Med Chem Lett. 2015;6:1010–4.
Lin L, Hutzen B, Zuo M, Ball S, Deangelis S, Foust E, et al. Novel STAT3 phosphorylation inhibitors exhibit potent growth-suppressive activity in pancreatic and breast cancer cells. Cancer Res. 2010;70:2445–54.
Miccoli A, Dhiani BA, Mehellou Y. Phosphotyrosine prodrugs: design, synthesis and anti-STAT3 activity of ISS-610 aryloxy triester phosphoramidate prodrugs. Medchemcomm. 2019;10:200–8.
Moon SY, Lee H, Kim S, Hong JH, Chun SH, Lee HY, et al. Inhibition of STAT3 enhances sensitivity to tamoxifen in tamoxifen-resistant breast cancer cells. BMC Cancer. 2021;21:931.
Al-Jomah N, Al-Mohanna FH, Aboussekhra A. Tocilizumab suppresses the pro-carcinogenic effects of breast cancer-associated fibroblasts through inhibition of the STAT3/AUF1 pathway. Carcinogenesis. 2021;42:1439–48.
Amin T, Viol F, Krause J, Fahl M, Eggers C, Awwad F, et al. Cancer associated fibroblasts induce proliferation and therapeutic resistance to everolimus in neuroendocrine tumors through STAT3 activation. Neuroendocrinology. 2022;
Hu G, Cheng P, Pan J, Wang S, Ding Q, Jiang Z, et al. An IL6-Adenosine Positive Feedback Loop between CD73+ γδTregs and CAFs Promotes Tumor Progression in Human Breast Cancer. Cancer Immunol Res. 2020;8:1273–86.
Zhang L, Qu J, Qi Y, Duan Y, Huang Y-W, Zhou Z, et al. EZH2 engages TGFβ signaling to promote breast cancer bone metastasis via integrin β1-FAK activation. Nat Commun. 2022;13:2543.
Xu M, Zhang T, Xia R, Wei Y, Wei X. Targeting the tumor stroma for cancer therapy. Mol Cancer. 2022;21:208.
Midwood KS, Chiquet M, Tucker RP, Orend G. Tenascin-C at a glance. J Cell Sci. 2016;129:4321–7.
Angel I, Pilo Kerman O, Rousso-Noori L, Friedmann-Morvinski D. Tenascin C promotes cancer cell plasticity in mesenchymal glioblastoma. Oncogene. 2020;39:6990–7004.
Yilmaz A, Loustau T, Salomé N, Poilil Surendran S, Li C, Tucker RP, et al. Advances on the roles of tenascin-C in cancer. J Cell Sci. 2022;135:jcs260244.
Yalcin F, Dzaye O, Xia S. Tenascin-C Function in Glioma: Immunomodulation and Beyond. Adv Exp Med Biol. 2020;1272:149–72.
Lowy CM, Oskarsson T. Tenascin C in metastasis: A view from the invasive front. Cell Adh Migr. 2015;9:112–24.
Murdamoothoo D, Sun Z, Yilmaz A, Riegel G, Abou-Faycal C, Deligne C, et al. Tenascin-C immobilizes infiltrating T lymphocytes through CXCL12 promoting breast cancer progression. EMBO Mol Med. 2021;13:e13270.
Li Z-L, Zhang H-L, Huang Y, Huang J-H, Sun P, Zhou N-N, et al. Autophagy deficiency promotes triple-negative breast cancer resistance to T cell-mediated cytotoxicity by blocking tenascin-C degradation. Nat Commun. 2020;11:3806.
Geleta B, Tout FS, Lim SC, Sahni S, Jansson PJ, Apte MV, et al. Targeting Wnt/tenascin C-mediated cross talk between pancreatic cancer cells and stellate cells via activation of the metastasis suppressor NDRG1. J Biol Chem. 2022;298:101608.
Kang X, Xu E, Wang X, Qian L, Yang Z, Yu H, et al. Tenascin-c knockdown suppresses vasculogenic mimicry of gastric cancer by inhibiting ERK- triggered EMT. Cell Death Dis. 2021;12:890.
Weigel C, Maczis MA, Palladino END, Green CD, Maceyka M, Guo C, et al. Sphingosine Kinase 2 in Stromal Fibroblasts Creates a Hospitable Tumor Microenvironment in Breast Cancer. Cancer Res. 2023;83:553–67.
Bonneaud TL, Lefebvre CC, Nocquet L, Basseville A, Roul J, Weber H, et al. Targeting of MCL-1 in breast cancer-associated fibroblasts reverses their myofibroblastic phenotype and pro-invasive properties. Cell Death Dis. 2022;13:787.
Zhang Y, Liu Q, Zhang X, Huang H, Tang S, Chai Y, et al. Recent advances in exosome-mediated nucleic acid delivery for cancer therapy. J Nanobiotechnology. 2022;20:279.
Chen B, Sang Y, Song X, Zhang D, Wang L, Zhao W, et al. Exosomal miR-500a-5p derived from cancer-associated fibroblasts promotes breast cancer cell proliferation and metastasis through targeting USP28. Theranostics. 2021;11:3932–47.
Kim JE, Kim BG, Jang Y, Kang S, Lee JH, Cho NH. The stromal loss of miR-4516 promotes the FOSL1-dependent proliferation and malignancy of triple negative breast cancer. Cancer Lett. 2020;469:256–65.
Al-Tweigeri T, AlRaouji NN, Tulbah A, Arafah M, Aboussekhra M, Al-Mohanna F, et al. High AUF1 level in stromal fibroblasts promotes carcinogenesis and chemoresistance and predicts unfavorable prognosis among locally advanced breast cancer patients. Breast Cancer Res. 2022;24:46.
Al-Ansari MM, Al-Saif M, Arafah M, Eldali AM, Tulbah A, Al-Tweigeri T, et al. Clinical and functional significance of tumor/stromal ATR expression in breast cancer patients. Breast Cancer Res. 2020;22:49.
Tanaka Y, Ohno T, Kadonaga T, Kidokoro Y, Wakahara M, Nosaka K, et al. Podoplanin expression in cancer-associated fibroblasts predicts unfavorable prognosis in node-negative breast cancer patients with hormone receptor-positive/HER2 - negative subtype. Breast Cancer. 2021;28:822–8.
Klein AP. Pancreatic cancer epidemiology: understanding the role of lifestyle and inherited risk factors. Nat Rev Gastroenterol Hepatol. 2021;18:493–502.
Krishnamurty AT, Shyer JA, Thai M, Gandham V, Buechler MB, Yang YA, et al. LRRC15+ myofibroblasts dictate the stromal setpoint to suppress tumour immunity. Nature. 2022;611:148–54.
Ray U, Pathoulas CL, Thirusangu P, Purcell JW, Kannan N, Shridhar V. Exploiting LRRC15 as a Novel Therapeutic Target in Cancer. Cancer Res. 2022;82:1675–81.
Purcell JW, Tanlimco SG, Hickson J, Fox M, Sho M, Durkin L, et al. LRRC15 Is a Novel Mesenchymal Protein and Stromal Target for Antibody-Drug Conjugates. Cancer Res. 2018;78:4059–72.
Sharbeen G, McCarroll JA, Akerman A, Kopecky C, Youkhana J, Kokkinos J, et al. Cancer-Associated Fibroblasts in Pancreatic Ductal Adenocarcinoma Determine Response to SLC7A11 Inhibition. Cancer Res. 2021;81:3461–79.
Koppula P, Zhuang L, Gan B. Cystine transporter SLC7A11/xCT in cancer: ferroptosis, nutrient dependency, and cancer therapy. Protein Cell. 2021;12:599–620.
Francescone R, Barbosa Vendramini-Costa D, Franco-Barraza J, Wagner J, Muir A, Lau AN, et al. Netrin G1 Promotes Pancreatic Tumorigenesis through Cancer-Associated Fibroblast-Driven Nutritional Support and Immunosuppression. Cancer Discov. 2021;11:446–79.
Ritchie S, Pereira BA, Vennin C, Timpson P. Targeting genetically-tuned CAFs in pancreatic cancer via perlecan manipulation. Expert Opin Ther Targets. 2020;24:171–4.
Kalscheuer S, Khanna V, Kim H, Li S, Sachdev D, DeCarlo A, et al. Discovery of HSPG2 (Perlecan) as a Therapeutic Target in Triple Negative Breast Cancer. Sci Rep. 2019;9:12492.
MacDonald A, Priess M, Curran J, Guess J, Farutin V, Oosterom I, et al. Necuparanib, A Multitargeting Heparan Sulfate Mimetic, Targets Tumor and Stromal Compartments in Pancreatic Cancer. Mol Cancer Ther. 2019;18:245–56.
Sun C, Bai M, Ke W, Wang X, Zhao X, Lu Z. The HSP90 inhibitor, XL888, enhanced cell apoptosis via downregulating STAT3 after insufficient radiofrequency ablation in hepatocellular carcinoma. Life Sci. 2021;282:119762.
Zhang Y, Ware MB, Zaidi MY, Ruggieri AN, Olson BM, Komar H, et al. Heat Shock Protein-90 Inhibition Alters Activation of Pancreatic Stellate Cells and Enhances the Efficacy of PD-1 Blockade in Pancreatic Cancer. Mol Cancer Ther. 2021;20:150–60.
Froeling FEM, Feig C, Chelala C, Dobson R, Mein CE, Tuveson DA, et al. Retinoic Acid-Induced Pancreatic Stellate Cell Quiescence Reduces Paracrine Wnt–β-Catenin Signaling to Slow Tumor Progression. Gastroenterology. 2011;141:1486-1497.e14.
Carapuça EF, Gemenetzidis E, Feig C, Bapiro TE, Williams MD, Wilson AS, et al. Anti-stromal treatment together with chemotherapy targets multiple signalling pathways in pancreatic adenocarcinoma: Stroma and cancer co-targeting. J Pathol. 2016;239:286–96.
Kocher HM, Basu B, Froeling FEM, Sarker D, Slater S, Carlin D, et al. Phase I clinical trial repurposing all-trans retinoic acid as a stromal targeting agent for pancreatic cancer. Nat Commun. 2020;11:4841.
Sherman MH, Yu RT, Engle DD, Ding N, Atkins AR, Tiriac H, et al. Vitamin D Receptor-Mediated Stromal Reprogramming Suppresses Pancreatitis and Enhances Pancreatic Cancer Therapy. Cell. 2014;159:80–93.
Feig C, Jones JO, Kraman M, Wells RJB, Deonarine A, Chan DS, et al. Targeting CXCL12 from FAP-expressing carcinoma-associated fibroblasts synergizes with anti-PD-L1 immunotherapy in pancreatic cancer. Proc Natl Acad Sci U S A. 2013;110:20212–7.
Fearon DT, Janowitz T. AMD3100/Plerixafor overcomes immune inhibition by the CXCL12-KRT19 coating on pancreatic and colorectal cancer cells. Br J Cancer. 2021;125:149–51.
Lin Y-N, Schmidt MO, Sharif GM, Vietsch EE, Kiliti AJ, Barefoot ME, et al. Impaired CXCL12 signaling contributes to resistance of pancreatic cancer subpopulations to T cell-mediated cytotoxicity. Oncoimmunology. 2022;11:2027136.
S T, E K, L D, A Y, W T, Y H, et al. Effect of tocopherol conjugation on polycation-mediated siRNA delivery to orthotopic pancreatic tumors. Biomaterials advances [Internet]. 2023 [cited 2023 Feb 12];145. Available from: https://pubmed.ncbi.nlm.nih.gov/36512927/ .
Y H, S T, W T, D V, C Z, Y X, et al. Polycation fluorination improves intraperitoneal siRNA delivery in metastatic pancreatic cancer. Journal of controlled release : official journal of the Controlled Release Society. 2021;333. Available from: https://pubmed.ncbi.nlm.nih.gov/33774121/ . Cited 2023 Feb 12.
Bockorny B, Semenisty V, Macarulla T, Borazanci E, Wolpin BM, Stemmer SM, et al. BL-8040, a CXCR4 antagonist, in combination with pembrolizumab and chemotherapy for pancreatic cancer: the COMBAT trial. Nat Med. 2020;26:878–85.
Bockorny B, Macarulla T, Semenisty V, Borazanci E, Feliu J, Ponz-Sarvise M, et al. Motixafortide and Pembrolizumab Combined to Nanoliposomal Irinotecan, Fluorouracil, and Folinic Acid in Metastatic Pancreatic Cancer: The COMBAT/KEYNOTE-202 Trial. Clin Cancer Res. 2021;27:5020–7.
Ghasemi K, Ghasemi K. MSX-122: Is an effective small molecule CXCR4 antagonist in cancer therapy? Int Immunopharmacol. 2022;108:108863.
Hu C, Xia R, Zhang X, Li T, Ye Y, Li G, et al. circFARP1 enables cancer-associated fibroblasts to promote gemcitabine resistance in pancreatic cancer via the LIF/STAT3 axis. Mol Cancer. 2022;21:24.
Xin M, Ji X, De La Cruz LK, Thareja S, Wang B. Strategies to target the Hedgehog signaling pathway for cancer therapy. Med Res Rev. 2018;38:870–913.
Bariwal J, Kumar V, Dong Y, Mahato RI. Design of Hedgehog pathway inhibitors for cancer treatment. Med Res Rev. 2019;39:1137–204.
Valenti G, Quinn HM, Heynen GJJE, Lan L, Holland JD, Vogel R, et al. Cancer Stem Cells Regulate Cancer-Associated Fibroblasts via Activation of Hedgehog Signaling in Mammary Gland Tumors. Cancer Res. 2017;77:2134–47.
D B, S F, L B, Uf W, C F-D-C, Al W, et al. Hedgehog signaling promotes angiogenesis directly and indirectly in pancreatic cancer. Angiogenesis. 2020;23. Available from: https://pubmed.ncbi.nlm.nih.gov/32444947/ . cited 2023 Feb 14.
Shan T, Chen S, Chen X, Lin WR, Li W, Ma J, et al. Prometastatic mechanisms of CAF-mediated EMT regulation in pancreatic cancer cells. Int J Oncol. 2017;50:121–8.
Girardi D, Barrichello A, Fernandes G, Pereira A. Targeting the Hedgehog Pathway in Cancer: Current Evidence and Future Perspectives. Cells. 2019;8:153.
Steele NG, Biffi G, Kemp SB, Zhang Y, Drouillard D, Syu L, et al. Inhibition of Hedgehog Signaling Alters Fibroblast Composition in Pancreatic Cancer. Clin Cancer Res. 2021;27:2023–37.
Herting CJ, Karpovsky I, Lesinski GB. The tumor microenvironment in pancreatic ductal adenocarcinoma: current perspectives and future directions. Cancer Metastasis Rev. 2021;40:675–89.
Ho WJ, Jaffee EM, Zheng L. The tumour microenvironment in pancreatic cancer — clinical challenges and opportunities. Nat Rev Clin Oncol. 2020;17:527–40.
Katoh M. Genomic testing, tumor microenvironment and targeted therapy of Hedgehog-related human cancers. Clin Sci (Lond). 2019;133:953–70.
Petrova E, Matevossian A, Resh MD. Hedgehog acyltransferase as a target in pancreatic ductal adenocarcinoma. Oncogene. 2015;34:263–8.
Ph S, Rl H, Hc L, Ly C, Yc W, Cc W, et al. NKX6–1 mediates cancer stem-like properties and regulates sonic hedgehog signaling in leiomyosarcoma. Journal of biomedical science. 2021;28. Available from: https://pubmed.ncbi.nlm.nih.gov/33906647/ . Cited 2023 Feb 16.
Khan MA, Srivastava SK, Zubair H, Patel GK, Arora S, Khushman M, et al. Co-targeting of CXCR4 and hedgehog pathways disrupts tumor-stromal crosstalk and improves chemotherapeutic efficacy in pancreatic cancer. J Biol Chem. 2020;295:8413–24.
Tarannum M, Holtzman K, Dréau D, Mukherjee P, Vivero-Escoto JL. Nanoparticle combination for precise stroma modulation and improved delivery for pancreatic cancer. J Control Release. 2022;347:425–34.
Pijnappel EN, Wassenaar NPM, Gurney-Champion OJ, Klaassen R, van der Lee K, Pleunis-van Empel MCH, et al. Phase I/II Study of LDE225 in Combination with Gemcitabine and Nab-Paclitaxel in Patients with Metastatic Pancreatic Cancer. Cancers (Basel). 2021;13:4869.
Kumar V, Donthireddy L, Marvel D, Condamine T, Wang F, Lavilla-Alonso S, et al. Cancer-Associated Fibroblasts Neutralize the Anti-tumor Effect of CSF1 Receptor Blockade by Inducing PMN-MDSC Infiltration of Tumors. Cancer Cell. 2017;32:654-668.e5.
Sano M, Ijichi H, Takahashi R, Miyabayashi K, Fujiwara H, Yamada T, et al. Blocking CXCLs-CXCR2 axis in tumor-stromal interactions contributes to survival in a mouse model of pancreatic ductal adenocarcinoma through reduced cell invasion/migration and a shift of immune-inflammatory microenvironment. Oncogenesis. 2019;8:8.
Fang L, Che Y, Zhang C, Huang J, Lei Y, Lu Z, et al. LAMC1 upregulation via TGFβ induces inflammatory cancer-associated fibroblasts in esophageal squamous cell carcinoma via NF-κB-CXCL1-STAT3. Mol Oncol. 2021;15:3125–46.
Saxena S, Molczyk C, Purohit A, Ehrhorn E, Goel P, Prajapati DR, et al. Differential expression profile of CXC-receptor-2 ligands as potential biomarkers in pancreatic ductal adenocarcinoma. Am J Cancer Res. 2022;12:68–90.
Koyama Y, Wang P, Liang S, Iwaisako K, Liu X, Xu J, et al. Mesothelin/mucin 16 signaling in activated portal fibroblasts regulates cholestatic liver fibrosis. J Clin Invest. 2017;127:1254–70.
Chen W, Hill H, Christie A, Kim MS, Holloman E, Pavia-Jimenez A, et al. Targeting renal cell carcinoma with a HIF-2 antagonist. Nature. 2016;539:112–7.
Cowman SJ, Koh MY. Revisiting the HIF switch in the tumor and its immune microenvironment. Trends Cancer. 2022;8:28–42.
Garcia Garcia CJ, Huang Y, Fuentes NR, Turner MC, Monberg ME, Lin D, et al. Stromal HIF2 Regulates Immune Suppression in the Pancreatic Cancer Microenvironment. Gastroenterology. 2022;162:2018–31.
Hsu T-S, Lin Y-L, Wang Y-A, Mo S-T, Chi P-Y, Lai AC-Y. HIF-2α is indispensable for regulatory T cell function. Nat Commun. 2020;11:5005.
Koikawa K, Kibe S, Suizu F, Sekino N, Kim N, Manz TD, et al. Targeting Pin1 renders pancreatic cancer eradicable by synergizing with immunochemotherapy. Cell. 2021;184:4753-4771.e27.
Liu J, Wang Y, Mu C, Li M, Li K, Li S, et al. Pancreatic tumor eradication via selective Pin1 inhibition in cancer-associated fibroblasts and T lymphocytes engagement. Nat Commun. 2022;13:4308.
Wong KM, Horton KJ, Coveler AL, Hingorani SR, Harris WP. Targeting the Tumor Stroma: the Biology and Clinical Development of Pegylated Recombinant Human Hyaluronidase (PEGPH20). Curr Oncol Rep. 2017;19:47.
Provenzano PP, Cuevas C, Chang AE, Goel VK, Von Hoff DD, Hingorani SR. Enzymatic Targeting of the Stroma Ablates Physical Barriers to Treatment of Pancreatic Ductal Adenocarcinoma. Cancer Cell. 2012;21:418–29.
Stylianopoulos T, Martin JD, Chauhan VP, Jain SR, Diop-Frimpong B, Bardeesy N, et al. Causes, consequences, and remedies for growth-induced solid stress in murine and human tumors. Proc Natl Acad Sci USA. 2012;109:15101–8.
Chauhan VP, Martin JD, Liu H, Lacorre DA, Jain SR, Kozin SV, et al. Angiotensin inhibition enhances drug delivery and potentiates chemotherapy by decompressing tumour blood vessels. Nat Commun. 2013;4:2516.
Murphy JE, Wo JY, Ryan DP, Clark JW, Jiang W, Yeap BY, et al. Total Neoadjuvant Therapy With FOLFIRINOX in Combination With Losartan Followed by Chemoradiotherapy for Locally Advanced Pancreatic Cancer: A Phase 2 Clinical Trial. JAMA Oncol. 2019;5:1020.
Jacobetz MA, Chan DS, Neesse A, Bapiro TE, Cook N, Frese KK, et al. Hyaluronan impairs vascular function and drug delivery in a mouse model of pancreatic cancer. Gut. 2013;62:112–20.
Hingorani SR, Harris WP, Beck JT, Berdov BA, Wagner SA, Pshevlotsky EM, et al. Phase Ib Study of PEGylated Recombinant Human Hyaluronidase and Gemcitabine in Patients with Advanced Pancreatic Cancer. Clin Cancer Res. 2016;22:2848–54.
Ramanathan RK, McDonough SL, Philip PA, Hingorani SR, Lacy J, Kortmansky JS, et al. Phase IB/II Randomized Study of FOLFIRINOX Plus Pegylated Recombinant Human Hyaluronidase Versus FOLFIRINOX Alone in Patients With Metastatic Pancreatic Adenocarcinoma: SWOG S1313. JCO. 2019;37:1062–9.
Hingorani SR, Zheng L, Bullock AJ, Seery TE, Harris WP, Sigal DS, et al. HALO 202: Randomized Phase II Study of PEGPH20 Plus Nab-Paclitaxel/Gemcitabine Versus Nab-Paclitaxel/Gemcitabine in Patients With Untreated. Metastatic Pancreatic Ductal Adenocarcinoma JCO. 2018;36:359–66.
Van Cutsem E, Tempero MA, Sigal D, Oh D-Y, Fazio N, Macarulla T, et al. Randomized Phase III Trial of Pegvorhyaluronidase Alfa With Nab-Paclitaxel Plus Gemcitabine for Patients With Hyaluronan-High Metastatic Pancreatic Adenocarcinoma. JCO. 2020;38:3185–94.
Brody H. Lung cancer. Nature. 2020;587:S7.
Kakarla S, Chow KK, Mata M, Shaffer DR, Song X-T, Wu M-F, et al. Antitumor Effects of Chimeric Receptor Engineered Human T Cells Directed to Tumor Stroma. Mol Ther. 2013;21:1611–20.
Wang L-CS, Lo A, Scholler J, Sun J, Majumdar RS, Kapoor V, et al. Targeting Fibroblast Activation Protein in Tumor Stroma with Chimeric Antigen Receptor T Cells Can Inhibit Tumor Growth and Augment Host Immunity without Severe Toxicity. Cancer Immunol Res. 2014;2:154--66.
Lo A, Wang L-CS, Scholler J, Monslow J, Avery D, Newick K, et al. Tumor-Promoting Desmoplasia Is Disrupted by Depleting FAP-Expressing Stromal Cells. Cancer Res. 2015;75:2800–10.
Hiltbrunner S, Britschgi C, Schuberth P, Bankel L, Nguyen-Kim TDL, Gulati P, et al. Local delivery of CAR T cells targeting fibroblast activation protein is safe in patients with pleural mesothelioma: first report of FAPME, a phase I clinical trial. Ann Oncol. 2021;32:120–1.
Jiang Z, Cheng L, Wu Z, Zhou L, Wang H, Hong Q, et al. Transforming primary human hepatocytes into hepatocellular carcinoma with genetically defined factors. EMBO Rep. 2022;23:e54275.
Lee IK, Noguera-Ortega E, Xiao Z, Todd L, Scholler J, Song D, et al. Monitoring Therapeutic Response to Anti-FAP CAR T Cells Using [18F]AlF-FAPI-74. Clin Cancer Res. 2022;28:5330–42.
Ebert LM, Yu W, Gargett T, Toubia J, Kollis PM, Tea MN, et al. Endothelial, pericyte and tumor cell expression in glioblastoma identifies fibroblast activation protein (FAP) as an excellent target for immunotherapy. Clin Transl Immunology. 2020;9:e1191.
Li F, Zhao S, Wei C, Hu Y, Xu T, Xin X, et al. Development of Nectin4/FAP-targeted CAR-T cells secreting IL-7, CCL19, and IL-12 for malignant solid tumors. Front Immunol. 2022;13:958082.
Borgonje PE, Andrews LM, Herder GJM, de Klerk JMH. Performance and Prospects of [68Ga]Ga-FAPI PET/CT Scans in Lung Cancer. Cancers (Basel). 2022;14:5566.
Shintani Y, Fujiwara A, Kimura T, Kawamura T, Funaki S, Minami M, et al. IL-6 Secreted from Cancer-Associated Fibroblasts Mediates Chemoresistance in NSCLC by Increasing Epithelial-Mesenchymal Transition Signaling. J Thorac Oncol. 2016;11:1482–92.
Sato R, Imamura K, Semba T, Tomita Y, Saeki S, Ikeda K, et al. TGFβ Signaling Activated by Cancer-Associated Fibroblasts Determines the Histological Signature of Lung Adenocarcinoma. Cancer Res. 2021;81:4751–65.
Y L, M M, M K, C X, J F, A L, et al. Simultaneous targeting of TGF-β/PD-L1 synergizes with radiotherapy by reprogramming the tumor microenvironment to overcome immune evasion. Cancer cell. 2021;39. Available from: https://pubmed.ncbi.nlm.nih.gov/34506739/ . Cited 2023 Mar 16.
Shi X, Luo J, Weigel KJ, Hall SC, Du D, Wu F, et al. Cancer-Associated Fibroblasts Facilitate Squamous Cell Carcinoma Lung Metastasis in Mice by Providing TGFβ-Mediated Cancer Stem Cell Niche. Front Cell Dev Biol. 2021;9:668164.
Kang JI, Kim DH, Sung KW, Shim SM, Cha-Molstad H, Soung NK, et al. p62-Induced Cancer-Associated Fibroblast Activation via the Nrf2-ATF6 Pathway Promotes Lung Tumorigenesis. Cancers (Basel). 2021;13:864.
Wang Y, Lan W, Xu M, Song J, Mao J, Li C, et al. Cancer-associated fibroblast-derived SDF-1 induces epithelial-mesenchymal transition of lung adenocarcinoma via CXCR4/β-catenin/PPARδ signalling. Cell Death Dis. 2021;12:214.
Huang W-C, Yadav VK, Cheng W-H, Wang C-H, Hsieh M-S, Huang T-Y, et al. The MEK/ERK/miR-21 Signaling Is Critical in Osimertinib Resistance in EGFR-Mutant Non-Small Cell Lung Cancer Cells. Cancers (Basel). 2021;13:6005.
Li H, Qiu L, Liu Q, Ma Z, Xie X, Luo Y, et al. Senescent Fibroblasts Generate a CAF Phenotype through the Stat3 Pathway. Genes (Basel). 2022;13:1579.
Reinfeld BI, Madden MZ, Wolf MM, Chytil A, Bader JE, Patterson AR, et al. Cell-programmed nutrient partitioning in the tumour microenvironment. Nature. 2021;593:282–8.
Dey P, Kimmelman AC, DePinho RA. Metabolic Codependencies in the Tumor Microenvironment. Cancer Discov. 2021;11:1067–81.
Liu T, Han C, Fang P, Ma Z, Wang X, Chen H, et al. Cancer-associated fibroblast-specific lncRNA LINC01614 enhances glutamine uptake in lung adenocarcinoma. J Hematol Oncol. 2022;15:141.
Lian G-Y, Wan Y, Mak TS-K, Wang Q-M, Zhang J, Chen J, et al. Self-carried nanodrug (SCND-SIS3): A targeted therapy for lung cancer with superior biocompatibility and immune boosting effects. Biomaterials. 2022;288:121730.
Hj K, K Y, K K, Yj L, S L, Sy A, et al. Reprogramming of cancer-associated fibroblasts by apoptotic cancer cells inhibits lung metastasis via Notch1-WISP-1 signaling. Cellular & molecular immunology. 2022;19. Available from: https://pubmed.ncbi.nlm.nih.gov/36241874/ . Cited 2023 Feb 28.
Dekker E, Tanis PJ, Vleugels JLA, Kasi PM, Wallace MB. Colorectal cancer. Lancet. 2019;394:1467–80.
Kamali Zonouzi S, Pezeshki PS, Razi S, Rezaei N. Cancer-associated fibroblasts in colorectal cancer. Clin Transl Oncol. 2022;24:757–69.
Aizawa T, Karasawa H, Funayama R, Shirota M, Suzuki T, Maeda S, et al. Cancer-associated fibroblasts secrete Wnt2 to promote cancer progression in colorectal cancer. Cancer Med. 2019;8:6370–82.
Kobayashi H, Gieniec KA, Lannagan TRM, Wang T, Asai N, Mizutani Y, et al. The Origin and Contribution of Cancer-Associated Fibroblasts in Colorectal Carcinogenesis. Gastroenterology. 2022;162:890–906.
Liu L, Zhang Z, Zhou L, Hu L, Yin C, Qing D, et al. Cancer associated fibroblasts-derived exosomes contribute to radioresistance through promoting colorectal cancer stem cells phenotype. Exp Cell Res. 2020;391:111956.
Yang M, Li D, Jiang Z, Li C, Ji S, Sun J, et al. TGF-β-Induced FLRT3 Attenuation Is Essential for Cancer-Associated Fibroblast-Mediated Epithelial-Mesenchymal Transition in Colorectal Cancer. Mol Cancer Res. 2022;20:1247–59.
Ji Q, Liu X, Han Z, Zhou L, Sui H, Yan L, et al. Resveratrol suppresses epithelial-to-mesenchymal transition in colorectal cancer through TGF-β1/Smads signaling pathway mediated Snail/E-cadherin expression. BMC Cancer. 2015;15:97.
Dana P, Thumrongsiri N, Tanyapanyachon P, Chonniyom W, Punnakitikashem P, Saengkrit N. Resveratrol Loaded Liposomes Disrupt Cancer Associated Fibroblast Communications within the Tumor Microenvironment to Inhibit Colorectal Cancer Aggressiveness. Nanomaterials (Basel). 2022;13:107.
Savio M, Ferraresi A, Corpina C, Vandenberghe S, Scarlata C, Sottile V, et al. Resveratrol and Its Analogue 4,4’-Dihydroxy-trans-stilbene Inhibit Lewis Lung Carcinoma Growth In Vivo through Apoptosis, Autophagy and Modulation of the Tumour Microenvironment in a Murine Model. Biomedicines. 2022;10:1784.
Suh J, Kim D-H, Surh Y-J. Resveratrol suppresses migration, invasion and stemness of human breast cancer cells by interfering with tumor-stromal cross-talk. Arch Biochem Biophys. 2018;643:62–71.
Vancauwenberghe E, Noyer L, Derouiche S, Lemonnier L, Gosset P, Sadofsky LR, et al. Activation of mutated TRPA1 ion channel by resveratrol in human prostate cancer associated fibroblasts (CAF). Mol Carcinog. 2017;56:1851–67.
Zhang J, Chen B, Li H, Wang Y, Liu X, Wong KY, et al. Cancer-associated fibroblasts potentiate colorectal cancer progression by crosstalk of the IGF2-IGF1R and Hippo-YAP1 signaling pathways. J Pathol. 2023;259:205–19.
Naktubtim C, Payuhakrit W, Uttarawichien T, Hassametto A, Suwannalert P. YAP, a novel target regulates F-actin rearrangement-associated CAFs transformation and promotes colorectal cancer cell progression. Biomed Pharmacother. 2022;155:113757.
Wu AT, Yeh Y-C, Huang Y-J, Mokgautsi N, Lawal B, Huang T-H. Gamma-mangostin isolated from garcinia mangostana suppresses colon carcinogenesis and stemness by downregulating the GSK3β/β-catenin/CDK6 cancer stem pathway. Phytomedicine. 2022;95:153797.
Heichler C, Schmied A, Enderle K, Scheibe K, Murawska M, Schmid B, et al. Targeting STAT3 Signaling in COL1+ Fibroblasts Controls Colitis-Associated Cancer in Mice. Cancers (Basel). 2022;14:1472.
Heichler C, Scheibe K, Schmied A, Geppert CI, Schmid B, Wirtz S, et al. STAT3 activation through IL-6/IL-11 in cancer-associated fibroblasts promotes colorectal tumour development and correlates with poor prognosis. Gut. 2020;69:1269–82.
Jonker DJ, Nott L, Yoshino T, Gill S, Shapiro J, Ohtsu A, et al. Napabucasin versus placebo in refractory advanced colorectal cancer: a randomised phase 3 trial. Lancet Gastroenterol Hepatol. 2018;3:263–70.
Zhou L, Li J, Tang Y, Yang M. Exosomal LncRNA LINC00659 transferred from cancer-associated fibroblasts promotes colorectal cancer cell progression via miR-342-3p/ANXA2 axis. J Transl Med. 2021;19:8.
Wang D, Wang X, Song Y, Si M, Sun Y, Liu X, et al. Exosomal miR-146a-5p and miR-155-5p promote CXCL12/CXCR7-induced metastasis of colorectal cancer by crosstalk with cancer-associated fibroblasts. Cell Death Dis. 2022;13:380.
Yang K, Zhang J, Bao C. Exosomal circEIF3K from cancer-associated fibroblast promotes colorectal cancer (CRC) progression via miR-214/PD-L1 axis. BMC Cancer. 2021;21:933.
Yang P, Zhang D, Wang T, Ji J, Jin C, Peng C, et al. CAF-derived exosomal WEE2-AS1 facilitates colorectal cancer progression via promoting degradation of MOB1A to inhibit the Hippo pathway. Cell Death Dis. 2022;13:796.
Chen X, Liu Y, Zhang Q, Liu B, Cheng Y, Zhang Y, et al. Exosomal miR-590-3p derived from cancer-associated fibroblasts confers radioresistance in colorectal cancer. Mol Ther Nucleic Acids. 2021;24:113–26.
Yin H, Yu S, Xie Y, Dai X, Dong M, Sheng C, et al. Cancer-associated fibroblasts-derived exosomes upregulate microRNA-135b-5p to promote colorectal cancer cell growth and angiogenesis by inhibiting thioredoxin-interacting protein. Cell Signal. 2021;84:110029.
Zhang H-W, Shi Y, Liu J-B, Wang H-M, Wang P-Y, Wu Z-J, et al. Cancer-associated fibroblast-derived exosomal microRNA-24-3p enhances colon cancer cell resistance to MTX by down-regulating CDX2/HEPH axis. J Cell Mol Med. 2021;25:3699–713.
Pan H, Pan J, Wu J. Development and validation of a cancer-associated fibroblast-derived lncRNA signature for predicting clinical outcomes in colorectal cancer. Front Immunol. 2022;13:934221.
Shen W, Yao P-A, Li W, Gu C, Gao T, Cao Y, et al. Cancer-associated fibroblast-targeted nanodrugs reshape colorectal tumor microenvironments to suppress tumor proliferation, metastasis and improve drug penetration. J Mater Chem B. 2023;11:1871–80.
Sanchez-Lopez E, Flashner-Abramson E, Shalapour S, Zhong Z, Taniguchi K, Levitzki A, et al. Targeting colorectal cancer via its microenvironment by inhibiting IGF-1 receptor-insulin receptor substrate and STAT3 signaling. Oncogene. 2016;35:2634–44.
Wang J, Delfarah A, Gelbach PE, Fong E, Macklin P, Mumenthaler SM, et al. Elucidating tumor-stromal metabolic crosstalk in colorectal cancer through integration of constraint-based models and LC-MS metabolomics. Metab Eng. 2022;69:175–87.
Makutani Y, Kawakami H, Tsujikawa T, Yoshimura K, Chiba Y, Ito A, et al. Contribution of MMP14-expressing cancer-associated fibroblasts in the tumor immune microenvironment to progression of colorectal cancer. Front Oncol. 2022;12:956270.
Zhang R, Liu F. Cancer-associated fibroblast-derived gene signatures predict radiotherapeutic survival in prostate cancer patients. J Transl Med. 2022;20:453.
Lang J, Zhao X, Qi Y, Zhang Y, Han X, Ding Y, et al. Reshaping Prostate Tumor Microenvironment To Suppress Metastasis via Cancer-Associated Fibroblast Inactivation with Peptide-Assembly-Based Nanosystem. ACS Nano. 2019;13:12357–71.
Kratochwil C, Flechsig P, Lindner T, Abderrahim L, Altmann A, Mier W, et al. 68Ga-FAPI PET/CT: Tracer Uptake in 28 Different Kinds of Cancer. J Nucl Med. 2019;60:801–5.
Kesch C, Yirga L, Dendl K, Handke A, Darr C, Krafft U, et al. High fibroblast-activation-protein expression in castration-resistant prostate cancer supports the use of FAPI-molecular theranostics. Eur J Nucl Med Mol Imaging. 2021;49:385–9.
Kakarla M, ChallaSivaKanaka S, Dufficy MF, Gil V, Filipovich Y, Vickman R, et al. Ephrin B Activate Src Family Kinases in Fibroblasts Inducing Stromal Remodeling in Prostate Cancer. Cancers (Basel). 2022;14:2336.
Heidegger I, Fotakis G, Offermann A, Goveia J, Daum S, Salcher S, et al. Comprehensive characterization of the prostate tumor microenvironment identifies CXCR4/CXCL12 crosstalk as a novel antiangiogenic therapeutic target in prostate cancer. Mol Cancer. 2022;21:132.
Xu Y, Ma J, Zheng Q, Wang Y, Hu M, Ma F, et al. MPSSS impairs the immunosuppressive function of cancer-associated fibroblasts via the TLR4-NF-κB pathway. Biosci Rep. 2019;39:BSR20182171.
Jia D, Zhou Z, Kwon O-J, Zhang L, Wei X, Zhang Y, et al. Stromal FOXF2 suppresses prostate cancer progression and metastasis by enhancing antitumor immunity. Nat Commun. 2022;13:6828.
Zhai X, Chen X, Wan Z, Ge M, Ding Y, Gu J, et al. Identification of the novel therapeutic targets and biomarkers associated of prostate cancer with cancer-associated fibroblasts (CAFs). Front Oncol. 2023;13:1136835.
Adisetiyo H, Liang M, Liao C-P, Jeong JH, Cohen MB, Roy-Burman P, et al. Dependence of castration-resistant prostate cancer (CRPC) stem cells on CRPC-associated fibroblasts. J Cell Physiol. 2014;229:1170–6.
Chen L, Wang Y-Y, Li D, Wang C, Wang S-Y, Shao S-H, et al. LMO2 upregulation due to AR deactivation in cancer-associated fibroblasts induces non-cell-autonomous growth of prostate cancer after androgen deprivation. Cancer Lett. 2021;503:138–50.
Kato M, Placencio-Hickok VR, Madhav A, Haldar S, Tripathi M, Billet S, et al. Heterogeneous cancer-associated fibroblast population potentiates neuroendocrine differentiation and castrate resistance in a CD105-dependent manner. Oncogene. 2019;38:716–30.
Zhang Z, Karthaus WR, Lee YS, Gao VR, Wu C, Russo JW, et al. Tumor Microenvironment-Derived NRG1 Promotes Antiandrogen Resistance in Prostate Cancer. Cancer Cell. 2020;38:279-296.e9.
Gil V, Miranda S, Riisnaes R, Gurel B, D’Ambrosio M, Vasciaveo A, et al. HER3 Is an Actionable Target in Advanced Prostate Cancer. Cancer Res. 2021;81:6207–18.
Cui D, Li J, Zhu Z, Berk M, Hardaway A, McManus J, et al. Cancer-associated fibroblast-secreted glucosamine alters the androgen biosynthesis program in prostate cancer via HSD3B1 upregulation. J Clin Invest. 2023;133:e161913.
Ippolito L, Morandi A, Taddei ML, Parri M, Comito G, Iscaro A, et al. Cancer-associated fibroblasts promote prostate cancer malignancy via metabolic rewiring and mitochondrial transfer. Oncogene. 2019;38:5339–55.
Martínez-Reyes I, Chandel NS. Cancer metabolism: looking forward. Nat Rev Cancer. 2021;21:669–80.
Ippolito L, Comito G, Parri M, Iozzo M, Duatti A, Virgilio F, et al. Lactate Rewires Lipid Metabolism and Sustains a Metabolic-Epigenetic Axis in Prostate Cancer. Cancer Res. 2022;82:1267–82.
Neuwirt H, Bouchal J, Kharaishvili G, Ploner C, Jöhrer K, Pitterl F, et al. Cancer-associated fibroblasts promote prostate tumor growth and progression through upregulation of cholesterol and steroid biosynthesis. Cell Commun Signal. 2020;18:11.
Kato M, Kurozumi A, Goto Y, Matsushita R, Okato A, Nishikawa R, et al. Regulation of metastasis-promoting LOXL2 gene expression by antitumor microRNAs in prostate cancer. J Hum Genet. 2017;62:123–32.
Xie P, Yu H, Wang F, Yan F, He X. Inhibition of LOXL2 Enhances the Radiosensitivity of Castration-Resistant Prostate Cancer Cells Associated with the Reversal of the EMT Process. Biomed Res Int. 2019;2019:4012590.
Comito G, Giannoni E, Segura CP, Barcellos-de-Souza P, Raspollini MR, Baroni G, et al. Cancer-associated fibroblasts and M2-polarized macrophages synergize during prostate carcinoma progression. Oncogene. 2014;33:2423–31.
Zhang R, Zong J, Peng Y, Shi J, Du X, Liu H, et al. GPR30 knockdown weakens the capacity of CAF in promoting prostate cancer cell invasion via reducing macrophage infiltration and M2 polarization. J Cell Biochem. 2021;
Lo JA, Fisher DE. The melanoma revolution: from UV carcinogenesis to a new era in therapeutics. Science. 2014;346:945–9.
Jenkins RW, Fisher DE. Treatment of Advanced Melanoma in 2020 and Beyond. J Invest Dermatol. 2021;141:23–31.
Capparelli C, Rosenbaum S, Berger AC, Aplin AE. Fibroblast-derived Neuregulin 1 Promotes Compensatory ErbB3 Receptor Signaling in Mutant BRAF Melanoma. J Biol Chem. 2015;290:24267–77.
Zhou L, Yang K, Randall Wickett R, Zhang Y. Dermal fibroblasts induce cell cycle arrest and block epithelial–mesenchymal transition to inhibit the early stage melanoma development. Cancer Med. 2016;5:1566–79.
Zhou L, Yang K, Dunaway S, Abdel-Malek Z, Andl T, Kadekaro AL, et al. Suppression of MAPK signaling in BRAF-activated PTEN-deficient melanoma by blocking β-catenin signaling in cancer-associated fibroblasts. Pigment Cell Melanoma Res. 2018;31:297–307.
Liu T, Zhou L, Xiao Y, Andl T, Zhang Y. BRAF Inhibitors Reprogram Cancer-Associated Fibroblasts to Drive Matrix Remodeling and Therapeutic Escape in Melanoma. Cancer Res. 2022;82:419–32.
Papaccio F, Kovacs D, Bellei B, Caputo S, Migliano E, Cota C, et al. Profiling Cancer-Associated Fibroblasts in Melanoma. Int J Mol Sci. 2021;22:7255.
Shelton M, Anene CA, Nsengimana J, Roberts W, Newton-Bishop J, Boyne JR. The role of CAF derived exosomal microRNAs in the tumour microenvironment of melanoma. Biochim Biophys Acta Rev Cancer. 2021;1875:188456.
Hu T, Hu J. Melanoma-derived exosomes induce reprogramming fibroblasts into cancer-associated fibroblasts via Gm26809 delivery. Cell Cycle. 2019;18:3085–94.
Zhou X, Yan T, Huang C, Xu Z, Wang L, Jiang E, et al. Melanoma cell-secreted exosomal miR-155-5p induce proangiogenic switch of cancer-associated fibroblasts via SOCS1/JAK2/STAT3 signaling pathway. J Exp Clin Cancer Res. 2018;37:242.
Anelli V, Mione M. Melanoma niche formation: it is all about melanosomes making CAFs. Pigment Cell Melanoma Res. 2017;30:8–10.
Dror S, Sander L, Schwartz H, Sheinboim D, Barzilai A, Dishon Y, et al. Melanoma miRNA trafficking controls tumour primary niche formation. Nat Cell Biol. 2016;18:1006–17.
Killock D. Cell signalling: Melanoma melanosomes shape the stromal niche. Nat Rev Clin Oncol. 2016;13:590–1.
Munir H, Jones JO, Janowitz T, Hoffmann M, Euler M, Martins CP, et al. Stromal-driven and Amyloid β-dependent induction of neutrophil extracellular traps modulates tumor growth. Nat Commun. 2021;12:683.
Song M, He J, Pan Q, Yang J, Zhao J, Zhang Y, et al. Cancer-Associated Fibroblast-Mediated Cellular Crosstalk Supports Hepatocellular Carcinoma Progression. Hepatology. 2021;73:1717–35.
Sun L, Wang Y, Wang L, Yao B, Chen T, Li Q, et al. Resolvin D1 prevents epithelial-mesenchymal transition and reduces the stemness features of hepatocellular carcinoma by inhibiting paracrine of cancer-associated fibroblast-derived COMP. J Exp Clin Cancer Res. 2019;38:170.
Sulciner ML, Serhan CN, Gilligan MM, Mudge DK, Chang J, Gartung A, et al. Resolvins suppress tumor growth and enhance cancer therapy. J Exp Med. 2018;215:115–40.
Mattoscio D, Isopi E, Lamolinara A, Patruno S, Medda A, De Cecco F, et al. Resolvin D1 reduces cancer growth stimulating a protective neutrophil-dependent recruitment of anti-tumor monocytes. J Exp Clin Cancer Res. 2021;40:129.
Eun JW, Yoon JH, Ahn HR, Kim S, Kim YB, Lim SB, et al. Cancer-associated fibroblast-derived secreted phosphoprotein 1 contributes to resistance of hepatocellular carcinoma to sorafenib and lenvatinib. Cancer Commun (Lond). 2023;43:455–79.
Grunberg N, Pevsner-Fischer M, Goshen-Lago T, Diment J, Stein Y, Lavon H, et al. Cancer-Associated Fibroblasts Promote Aggressive Gastric Cancer Phenotypes via Heat Shock Factor 1-Mediated Secretion of Extracellular Vesicles. Cancer Res. 2021;81:1639–53.
Yang Y, Ma Y, Yan S, Wang P, Hu J, Chen S, et al. CAF promotes chemoresistance through NRP2 in gastric cancer. Gastric Cancer. 2022;25:503–14.
Ham I-H, Wang L, Lee D, Woo J, Kim TH, Jeong HY, et al. Curcumin inhibits the cancer-associated fibroblast-derived chemoresistance of gastric cancer through the suppression of the JAK/STAT3 signaling pathway. Int J Oncol. 2022;61:85.
Lu Y, Jin Z, Hou J, Wu X, Yu Z, Yao L, et al. Calponin 1 increases cancer-associated fibroblasts-mediated matrix stiffness to promote chemoresistance in gastric cancer. Matrix Biol. 2023;115:1–15.
Akiyama T, Yasuda T, Uchihara T, Yasuda-Yoshihara N, Tan BJY, Yonemura A, et al. Stromal Reprogramming through Dual PDGFRα/β Blockade Boosts the Efficacy of Anti-PD-1 Immunotherapy in Fibrotic Tumors. Cancer Res. 2023;83:753–70.
Yang K-D, Wang Y, Zhang F, Li Q-L, Luo B-H, Feng D-Y, et al. CAF-derived midkine promotes EMT and cisplatin resistance by upregulating lncRNA ST7-AS1 in gastric cancer. Mol Cell Biochem. 2022;477:2493–505.
Ma Z, Li X, Mao Y, Wei C, Huang Z, Li G, et al. Interferon-dependent SLC14A1+ cancer-associated fibroblasts promote cancer stemness via WNT5A in bladder cancer. Cancer Cell. 2022;40:1550-1565.e7.
Zhuang J, Shen L, Li M, Sun J, Hao J, Li J, et al. Cancer-associated fibroblast-derived miR-146a-5p generates a niche that promotes bladder cancer stemness and chemoresistance. Cancer Res. 2023;83(10):1611–27.
Jain S, Rick JW, Joshi RS, Beniwal A, Spatz J, Gill S, et al. Single-cell RNA sequencing and spatial transcriptomics reveal cancer-associated fibroblasts in glioblastoma with protumoral effects. J Clin Invest. 2023;133:e147087.
Alghamri MS, Banerjee K, Mujeeb AA, Mauser A, Taher A, Thalla R, et al. Systemic Delivery of an Adjuvant CXCR4-CXCL12 Signaling Inhibitor Encapsulated in Synthetic Protein Nanoparticles for Glioma Immunotherapy. ACS Nano. 2022;16:8729–50.
Hou Y-J, Li D, Wang W, Mao L, Fu X, Sun B, et al. NT157 inhibits cell proliferation and sensitizes glioma cells to TRAIL-induced apoptosis by up-regulating DR5 expression. Biomed Pharmacother. 2022;153:113502.
Iida T, Mizutani Y, Esaki N, Ponik SM, Burkel BM, Weng L, et al. Pharmacologic conversion of cancer-associated fibroblasts from a protumor phenotype to an antitumor phenotype improves the sensitivity of pancreatic cancer to chemotherapeutics. Oncogene. 2022;41:2764–77.
Download references
Acknowledgements
The authors appreciate Figdraw and Biorender for supporting the material in graphics in this article.
This work was supported by the National Natural Science Foundation of China (NO. 82073893), Hunan Provincial Natural Science Foundation of China (NO.2022JJ20095), Hunan Provincial Health Committee Foundation of China (NO.202204044869), China Postdoctoral Science Foundation (2023MD734131), Chongqing Postdoctoral Science Foundation (CSTB2023NSCQBHX0002), Kuanren Talents Program of the second affiliated hospital of Chongqing Medical University, Chongqing Scientific and Health Joint Medical Research Project (2020GDRC021).
Author information
Hao Zhang and Xinghai Yue contributed equally to this work.
Authors and Affiliations
Department of Neurosurgery, The Second Affiliated Hospital, Chongqing Medical University, Chongqing, China
Hao Zhang, Xinghai Yue, Zhe Chen, Peng Luo & Guodong Liu
Department of Urology, The Second Affiliated Hospital, Chongqing Medical University, Chongqing, China
Xinghai Yue & Qing Jiang
Department of Neurosurgery, Central Hospital of Zhuzhou, Zhuzhou, China
Department of Oncology, Xiangya Hospital, Central South University, Changsha, China
College of Life Science and Technology, Huazhong University of Science and Technology, Wuhan, China
Department of Interventional Radiology, The First Affiliated Hospital of Zhengzhou University, Zhengzhou, China
Department of Laboratory Medicine, The Second Affiliated Hospital, Chongqing Medical University, Chongqing, China
Liping Yang
Department of Neurosurgery, Xiangya Hospital, Central South University, Changsha, China
National Clinical Research Center for Geriatric Disorders, Xiangya Hospital, Central South University, Changsha, China
Department of Oncology, Zhujiang Hospital, Southern Medical University, Guangzhou, China
You can also search for this author in PubMed Google Scholar
Contributions
XY and HZ designed and drafted the manuscript. CL, ZC, WW, NZ, ZL, LY, QJ, PL, QC, and GL revised the manuscript. PL, QC, and GL supervised the manuscript and provided funding support. All authors have read and approved the final manuscript.
Corresponding authors
Correspondence to Quan Cheng , Peng Luo or Guodong Liu .
Ethics declarations
Ethics approval and consent to participate, consent for publication.
All authors have agreed on the contents of the manuscript.
Competing interests
The authors declare no competing interests.
Additional information
Publisher’s note.
Springer Nature remains neutral with regard to jurisdictional claims in published maps and institutional affiliations.
Rights and permissions
Open Access This article is licensed under a Creative Commons Attribution 4.0 International License, which permits use, sharing, adaptation, distribution and reproduction in any medium or format, as long as you give appropriate credit to the original author(s) and the source, provide a link to the Creative Commons licence, and indicate if changes were made. The images or other third party material in this article are included in the article's Creative Commons licence, unless indicated otherwise in a credit line to the material. If material is not included in the article's Creative Commons licence and your intended use is not permitted by statutory regulation or exceeds the permitted use, you will need to obtain permission directly from the copyright holder. To view a copy of this licence, visit http://creativecommons.org/licenses/by/4.0/ . The Creative Commons Public Domain Dedication waiver ( http://creativecommons.org/publicdomain/zero/1.0/ ) applies to the data made available in this article, unless otherwise stated in a credit line to the data.
Reprints and permissions
About this article
Cite this article.
Zhang, H., Yue, X., Chen, Z. et al. Define cancer-associated fibroblasts (CAFs) in the tumor microenvironment: new opportunities in cancer immunotherapy and advances in clinical trials. Mol Cancer 22 , 159 (2023). https://doi.org/10.1186/s12943-023-01860-5
Download citation
Received : 19 June 2023
Accepted : 13 September 2023
Published : 02 October 2023
DOI : https://doi.org/10.1186/s12943-023-01860-5
Share this article
Anyone you share the following link with will be able to read this content:
Sorry, a shareable link is not currently available for this article.
Provided by the Springer Nature SharedIt content-sharing initiative
- Clinical trial
- Microenvironment
- Immunotherapy
Molecular Cancer
ISSN: 1476-4598
- General enquiries: [email protected]
- Open access
- Published: 26 August 2024
Tumoroids, a valid preclinical screening platform for monitoring cancer angiogenesis
- Zahra Abbasi-Malati 1 na1 ,
- Parisa Khanicheragh 2 na1 ,
- Maryam Taghavi Narmi 2 ,
- Narges Mardi 3 ,
- Nafiseh Didar Khosrowshahi 4 ,
- Amirataollah Hiradfar 5 ,
- Aysa Rezabakhsh 6 ,
- Fatemeh Sadeghsoltani 2 ,
- Somayyeh Rashidi 7 ,
- Sara Aghakhani Chegeni 2 ,
- Golbarg Roozbahani 8 &
- Reza Rahbarghazi ORCID: orcid.org/0000-0003-3864-9166 2 , 9
Stem Cell Research & Therapy volume 15 , Article number: 267 ( 2024 ) Cite this article
28 Accesses
Metrics details
In recent years, biologists and clinicians have witnessed prominent advances in in vitro 3D culture techniques related to biomimetic human/animal tissue analogs. Numerous data have confirmed that unicellular and multicellular (tumoroids) tumor spheroids with dense native cells in certain matrices are sensitive and valid analytical tools for drug screening, cancer cell dynamic growth, behavior, etc. in laboratory settings. Angiogenesis/vascularization is a very critical biological phenomenon to support oxygen and nutrients to tumor cells within the deep layer of solid masses. It has been shown that endothelial cell (EC)-incorporated or -free spheroid/tumoroid systems provide a relatively reliable biological platform for monitoring the formation of nascent blood vessels in micron/micrometer scales. Besides, the paracrine angiogenic activity of cells within the spheroid/tumoroid systems can be monitored after being treated with different therapeutic approaches. Here, we aimed to collect recent advances and findings related to the monitoring of cancer angiogenesis using unicellular and multicellular tumor spheroids. Vascularized spheroids/tumoroids can help us in the elucidation of mechanisms related to cancer formation, development, and metastasis by monitoring the main influencing factors.
Introduction
Inside the body, cells reside in a specific three-dimensional (3D) microenvironment which is known as a niche. The close interaction between cells and the environment within the defined 3D space can regulate each cell's morphology and function [ 1 ]. From the past to the present time, cell culture has been widely used to understand the participation of various molecular pathways, cell behaviors, and the mechanism of diseases and pathological conditions [ 2 ]. In this regard, the conventional two-dimensional (2D) culture system is a basic method for the cultivation, expansion, and conduction of several experiments in most laboratories [ 3 ]. As a common belief, the 2D culture system is a relatively low-cost and friendly-use in vitro model for large-scale production and harvesting of human and animal cells [ 2 ]. In this system, adherent cells are directly attached to a plastic surface predominantly composed of polystyrene-based materials [ 4 ]. While the non-adherent cells are expanded without the necessity to attach to the bottom of culturing flasks [ 5 ]. Despite these advantages, several issues and limitations restrict the generalization of in vitro data to in vivo conditions. It is postulated that 2D culture systems could not completely reflect the in vivo conditions because of the lack of accurate tissue model structures, cellular heterogeneity, and real-time pathological changes [ 6 ]. Generally, the monolayer cell culture systems produce flattened cells with unlimited access to micro-, macro-elements, metabolites, signaling molecules, and growth factors, leading to uncontrolled cell proliferation and expansion, and loss of specified morphologies and functions [ 7 , 8 ]. Of course, the subcellular localization of organelles, paracrine activity, and stimulation of certain signaling pathways are also changed in cells cultured in 2D systems [ 9 , 10 ]. Under such conditions, the proteomic, metabolic, and genomic profiles of cells are also changed compared to cells of the same lineages inside the body [ 11 ]. Due to the easy distribution of soluble factors in 2D culture systems, even trivial concentrations of therapeutics can evenly affect the cultured cells which is not comparable to the in vivo conditions while in the in vivo microenvironment, there is a competition between the cells to access nutrients and other signaling molecules [ 12 ]. Besides, diffusible molecules are delivered to various tissues in a concentration gradient manner [ 13 ]. The absence of extracellular matrix (ECM), multiple signals, and mechanical cues inhibit or blunt the activity of certain molecular effectors inside the cells, resulting in low-rate predictability [ 14 ]. High-rate proliferation and expansion of cells in 2D culture systems can result in the existence of numerous dead cells with diverse waste products which can affect the physiology of co-expanded cells [ 15 , 16 , 17 ]. To overcome these limitations, 3D in vitro cell culture systems have been advanced for basic and pre-clinical cancer research [ 18 ]. In this regard, Petersen and Bissell presented the 3D organotypic structures to mimic healthy and cancerous breast tissue niches [ 19 ]. It is believed that in vitro organoid systems can in part, but not completely, provide the key elements of in vivo conditions via the regulation of cell morphologies, juxtacrine and paracrine activities, and cell-to-ECM interaction [ 20 ]. Using various supporting ECM components at different ratios, organoid (tumoroid) microstructure can be developed similar to the native tissues [ 18 ]. Despite the existence of different limitations, 3D culture settings provide valuable information about cell behavior for clinical use compared to 2D culture settings [ 21 , 22 ].
As above-mentioned, the development of optimized biomimetic culture systems with high similarity to in vivo conditions can yield more valid and comparable data [ 23 ]. To date, several 3D culture systems have been developed to recapitulate the physiological and pathological conditions (Table 1 ). Among several 3D culture systems, organoids/spheroids are engineered in vitro micro/macrostructures composed of stem cells, cancer cells, and/or mature cells and have been used for theranostics, large‐scale drug screening, hereditary diseases, and personalized medicine purposes (Table 2 ) [ 24 , 25 ]. In this review article, the recent advances in 3D culture systems with a focus on organoids/spheroids will be discussed in terms of stem cell biology and tumor cells.
Different organoid/spheroid systems in biomedical fields
Terms and general features.
The advent and development of 3D culture systems have helped biologists in the conduction of numerous animal-free studies for basic biology and clinical research [ 26 ]. Irrespective of ethical issues, data from animal studies are relatively inefficient in understanding the physiopathological conditions of human counterparts and could not be completely applied to clinical settings [ 27 ]. The cost of drugs and therapeutic applications is high on different animal models. The reproducibility and reliability analyses on animals are under question as different animal strains are treated using rigorous protocols and conditions [ 28 ]. Of note, most of the drugs examined in animals fail to yield relatively similar responses in human counterparts because of differences in animal models, strain, dose of therapeutics, and administration route [ 28 ]. To be specific, organoids and spheroids are 3D miniaturized tissue structures with either stem cells, mature cells, or even cancer cells to recapitulate the in vivo-like microenvironments and efficient pre-clinical platforms for drug screening and validation [ 1 ]. Carcinogenesis and promotion of anaplastic changes coincide with the activation of several sequential processes that provoke cancer cells and non-cancer cells into the tumor stroma [ 29 ]. To date, several techniques and approaches have been used for the fabrication of organoids/spheroids, and other 3D culture systems (Table 1 and Fig. 1 ). Data have confirmed that cells maintained and expanded within the organoid/spheroid/tumoroid system exhibited relatively similar patterns of in vivo dynamic growth and response to therapeutics compared to conventional 2D culture systems [ 30 ]. Progress and advancement in 3D culture systems like organoids have led to the compensation of gaps and shortcomings between the 2D culture system and animal models [ 16 , 17 ]. In this scenario, the 3D culture system exhibits more valuable data about the physiology of cells because of reciprocal cell-to-cell and cell-to-ECM interaction [ 16 , 17 ].
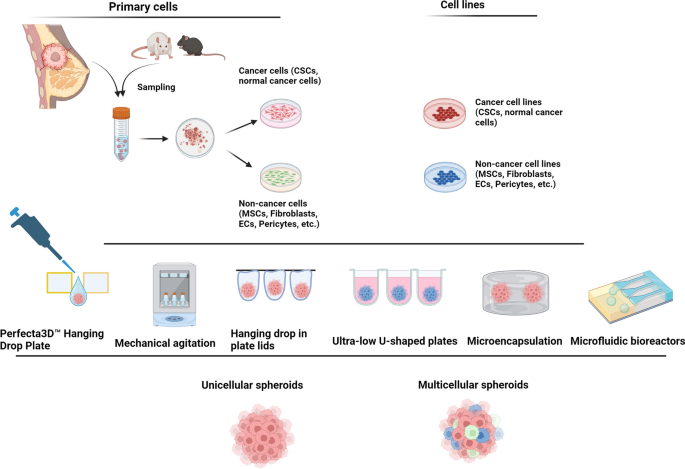
Different approaches are used for the development of unicellular (homotypic) and multicellular (heterotypic) tumor spheroids in in vitro conditions. Several studies have been performed to generate tumor spheroids by using different cells such as freshly isolated or cultured primary cells, and cancer and non-cancer cell lines. The cancer cell aggregate is composed of single cancer cells (spheroids) or different cell lineages (multicellular spheroids or tumoroids) using the supporting matrix to maintain the cells within certain sizes. Designed by using BioRender's web-based software
In cancer biology, several tumoroid models have been used for the evaluation of therapeutics as follows; 3D tumor cell culture, 3D tumor slice, patient-derived organoids, vascularized tumoroid, spheroid, and organoid culture models [ 31 , 32 , 33 , 34 , 35 , 36 , 37 ]. Most often there is an overlap between the terms spheroids and organoids concerning cellular sources and generation procedure [ 33 ]. Spheroids have less complexity and are common 3D structures for preliminary monitoring of anti-cancer drugs while organoids have higher similarities in terms of genetic patterns and histological features to the source tissues and tumors [ 1 ]. In the context of cancers, assembling disassociated tumor cells using suitable ECM components generates organoids which are known as tumoroids [ 31 ]. It has been proposed that 3D culture systems like organoids are valid tools to simulate tiny tumor masses using several cell types with relatively in vivo-like growth and treatment patterns [ 16 , 17 ]. To the best of our knowledge, unicellular tumor spheroids have been extensively utilized for cancer cell biology, invasion, and drug screening. However, they do not accurately mimic the intricate biological and clinical characteristics of primary tumor tissues, thus restricting their effectiveness in predicting individualized responses to chemotherapy. In contrast, organoids (known also tumoroids) exhibited more valuable tools for drug screening, disease modeling, and personalized medicine. Tumor organoids can be developed from primary patient samples within a defined 3D framework. It is thought that tumoroids have relatively structural and functional cues like in vivo tumor masses, with a reliable platform for clinical decision-making [ 38 ]. Unlike tumoroids, single-cell spheroids lack cell polarity, heterogenicity, and complexity and function of cancer cells within the cancer masses. Thus, organoids reflect more accurately the cellular diversity and physiological roles found in organs [ 39 ]. Besides, in stem cell research, organoids composed of tissue-specific stem cells with other specific cell lineages have been used to mimic the architecture and function of the target tissues [ 40 ]. Stem cell organoids are effective in vitro modeling tools in the establishment of studies related to developmental processes (organogenesis), regenerative defects, and certain pathophysiological conditions. Retention inside the defined microenvironments helps the stem cells to preserve their stemness while it simultaneously helps us to use naïve or genetically manipulated stem cells for specific regenerative purposes [ 40 ].
For conventional organoid/tumoroid development, three main elements including broad-ranging cells (either homogenous or heterogeneous cell type), supporting scaffolds, and endogenous/exogenous signaling molecules can be modulated according to the purpose and objective of the study [ 1 ]. In several studies, both natural and synthetic substrates have been used for the generation of organoids/tumoroids to provide a platform for cell attachment, proliferation, and juxtacrine within a 3D structure [ 41 ] (Table 1 ). For generation of organoid/tumoroid structure using natural ECM components, the existence of specific motifs such as Arg-Gly-Asp (RGD) can be useful in providing an in vivo-like microenvironment. Despite the several advantages (toxicity↓, biocompatibility↑, bioactivity↑, and biodegradability↑) related to the application of natural substrates, the final organoids/tumoroids lack appropriate mechanical stability and integrity in response to the changes of certain parameters such as pH and incubation temperature [ 42 ]. In line with these comments, the addition of synthetic substrates such as polyurethane (PU)-, polyethylene glycol (PEG)-, poly (lactic-co-glycolic acid) (PLGA)-based composites can yield organoids/tumoroids with higher mechanical properties and resistance to environmental parameters. However, the lack of essential bioactive motifs like RGD, etc. restricts their bulk application in organoid/tumoroid fabrication [ 43 ].
Mechanisms related to tumoroid formation
Due to the complexity of the tumor microenvironment (TME) and the involvement of different cell types, it seems that an intricate molecular network is involved in the formation of tumor colonies [ 44 ]. Like in vivo conditions, in vitro protocols should be performed with a focus on the stimulation of such molecular interactions and specific cell behavior to promote cell aggregation within distinct dimensions and geometries. In a common belief, the loosely cell-to-cell connection is promoted in in vitro conditions mainly via the attachment of integrins and cadherins to ECM components, leading to the formation of preliminary cell aggregates [ 45 , 46 ]. The initial spheroid units undergo compaction via the maintenance of homotypic cadherin binding [ 45 ]. By the promotion of homotypic (homophilic) cadherin interaction, cells are juxtaposed in contact areas while the surface tension is also diminished [ 47 ]. In this regard, E and N-cadherins are the most important cadherin types and they participate in homophilic cell-to-cell attachment [ 48 ]. However, the possibility of heterophilic interaction between N and E-cadherins is also possible via physical interactions between the cadherins ectodomains [ 48 ]. Upon induction of cadherin-to-cadherin interaction, intracellular actomyosin contractility is stimulated, resulting in cell aggregate morphogenesis [ 49 ]. Of course, the type and level of cadherins are associated with the cell lineage. For instance, E-cadherin is actively involved in juxtacrine interaction between the cancer cells rather than cancer stem cells (CSCs). Powan and co-workers indicated that the lack of cell-to-cell connection via cadherin can increase the possibility of apoptosis (p53↑, phosphorylated FAK↓), leading to loss of integrity in tumor cell aggregates [ 50 ]. Despite the existence of heterophilic cadherin interaction between the CSC N-cadherin and non-CSC E-cadherin, it seems that this interaction is not highly stable when compared to the homophilic E-cadherin-to-E-cadherin connection. Besides, the conversion of E-cadherin to N-cadherin during the anaplastic changes can reduce the possibility of colony formation and increase the metastatic behavior, leading to the disintegration of multicellular components within the tumoroid systems [ 51 , 52 ]. Previous data showed the co-precipitation of cytoskeletal proteins like actin with N-cadherin, indicating the ability of N-cadherin to modulate specific cell morphologies between the juxtaposed cells [ 46 ]. As above-mentioned, the activity of ECM components along with cell surface adherens can promote the integrity of cell-to-cell junction and thus tumoroid stability. For instance, fibronectin with fibrillar strands can recruit tensin, and α5β1 integrins and strengthen the physical contacts between the cells. The loss of fibronectin can result in N-cadherin ligation recruited α5β1 integrins, tensin, and β-catenin [ 46 ]. These data show that cells tend to preserve their juxtacrine interactions using different mechanisms even in the absence of certain ECM components.
Of note, the application of several cells within the tumoroid system can stimulate several cell-to-cell junction pathways. It has been shown that CAFs can secret different fibroblast growth factor members within the ovarian tumor mass [ 53 ]. In the presence of FGF-2, the stability of VE-cadherin increases between the juxtaposed ECs via controlling SHP2 [ 54 ]. These features can promote blood support into tumor parenchyma and facilitate tumor cell growth and expansion. According to these data, one can hypothesize that the integrity of cells via juxtacrine interaction can be induced via the application of multiple cells within the tumoroid system rather than of unicellular units. Within the tumor parenchyma, ECM is remodeled with the balances between the production and degradation. For example, several cell types such as tumor associate macrophages (TAMs) and CAFs can release various MMPs (MMP2, 7, 9, and 12) along with cytokines such as VEGF and FGF-2 into tumor parenchyma, leading to bulk remodeling of ECM and angiogenesis [ 55 ]. Collagen is the most abundant ECM component with the potential to induce cancer fibrosis. The mutual tumor cell-collagen interaction is done via the engagement of several signaling pathways such as transforming growth factor-β (TGF-β)/Smad axis, etc. Collagen fibers in proximity to epithelial cells generate a stiff fibrotic layer via reciprocal interaction between the Smad4 and phosphorylated myosin light chain 2 [ 56 ]. Inside tumor parenchyma, several collagen types are produced with diverse biological activities. The orientation, alignment, and stroma stiffness are related to the size and fiber type of collagen in which the long collagen fibers are closely aligned to each other and stabilize cell localization and organization. There is a physical contact between cell surface integrins α ( 1 , 2 , 3 , 4 , 10 , 11 )/β 1 subunits with ECM collagen fibers. Unlike long collagen fibers, short fibers can increase the permeability of tumor niches [ 57 ]. In terms of angiogenesis, it was suggested that collagen stiffness can influence microvascular integrity and development. Of note, there is an inverse relationship between the microvascular network distribution and collagen fiber density. On the other hand, the increase of inter-collagen fibril branching and spatial arrangement (especially type IV collagen) promotes vascular density and number [ 57 ]. In in vitro conditions, the application of collagen with basal membrane components takes approximately five days to generate relatively compact cell aggregates with certain viscoelastic behavior [ 58 ]. Therefore, the existence of supporting ECM can support simultaneously reciprocal cell-to-cell and cell-to-ECM interactions.
Tumoroids/spheroids and angiogenesis potential
Tumor angiogenesis.
It has been indicated that angiogenesis or neo-vascularization is a critical phenomenon for the development, and expansion of tumor cells within the solid cancer parenchyma [ 59 ]. Angiogenesis is the formation of new blood vessels from the pre-existing vascular network while vascularization is the phenomenon of in situ differentiation and expansion of vascular units with the participation of endothelial progenitor cells (EPCs) [ 60 ]. Angiogenesis with different signaling pathways, and cell components, especially endothelial cells (ECs) supports blood and oxygen demand to cells exposed to hypoxic conditions [ 60 ]. Considering the urgent need for establishing rapid, reproducible, and valid in vitro cancer angiogenesis models for the evaluation of various pro-, and anti-angiogenesis compounds, it seems that the selection of appropriate assays such as tumoroid/spheroid-based assays can help us to understand angiogenesis mechanisms better than conventional cell-based assays [ 61 ]. Most commonly used in vitro angiogenesis assays are based on the culture and expansion of ECs on the plastic surface or certain substrates (Table 3 ) [ 62 ]. Notably, 2D culture settings and numerous 3D culture assays can lead to the loss of typical function and phenotype of ECs [ 63 ]. Based on previously published data, cultured ECs in 2D culture systems exhibited prominent changes in karyotype, cell-surface antigens, and dynamic growth activity [ 64 ]. To achieve suitable angiogenesis potential, ECs must be in a quiescent state to stimulate the juxtaposition of several vascular cells with simultaneous activation of certain pro-angiogenesis factors [ 65 ]. Of note, there is no 2D gold-standard and definite angiogenesis assay for the detection of cellular and molecular events [ 65 ]. Thus, more angiogenesis-related assays are mandatory to achieve reliable results. It should not be forgotten that most assays use ECs as the only cellular source for angiogenesis assessment while different cell lineages with a high degree of heterogeneity (either structural or functional diversity) participate in blood vessel formation [ 66 ]. Noteworthy, the shape and size of ECs, and the complexity of junctions are significantly altered in 2D culture platforms compared to the in vivo conditions [ 64 ]. Commensurate with these descriptions, the development of novel angiogenesis protocols is highly recommended. To date, several 3D assays based on tumoroid/spheroid generation have been conducted to evaluate the angiogenesis in different tumor cells (Table 4 ) [ 67 ]. As mentioned above, it is thought that unicellular spheroids and multicellular tumoroids are valid avascular units and can mimic relatively in vivo cytoarchitectural features compared to the 2D culture systems.
Impact of hypoxia on angiogenesis
Inside the body, solid tumor cells are exposed to hypoxia when O 2 levels drop below 21% which can worsen the prognosis of cancer individuals [ 68 ]. Due to micron-size dimensions, tumoroids/spheroids can provide diffusional limitations and radial gradients in terms of O 2 , nutrients, micro- and macroelements, and waste byproducts [ 69 ]. Cells located at the deeper layers of organoid/tumoroid microstructures are more prone to hypoxic changes because of an imbalance between O 2 consumption and the limited distribution of nutrients [ 70 ]. It has been indicated that hypoxia is a potent angiogenesis stimulator via the regulation of several pro-angiogenic pathways. Besides the critical role of angiogenesis factors in different aspects of EC biology, hypoxic conditions can also influence vascular cell arrangement, and functions [ 68 ]. In response to hypoxic conditions, the activation of hypoxia-inducible factors (HIFs) can per se switch on the expression of multiple angiogenesis factors, leading to angiogenesis transition via direct interaction with cognate receptors on tumor cells, ECs, and other vascular cells [ 71 ]. HIFs consist of HIF-1α, -1β, -2α, and -3α with the potential (especially HIF-1α) to simultaneously alter the transcription of other genes such as vascular endothelial growth factor (VEGF), and erythropoietin following the attachment to hypoxia response elements (HREs) [ 72 ]. HIF-1α is inhibited via factor inhibiting HIF (FIH), and prolyl-hydroxylases (PHDs) when O 2 contents return to basal levels. In contrast, these factors (PHDs, and FIH) are inactive under hypoxic conditions which in turn promote the translocation of HIF-α into the nucleus and its interaction with HIF-β [ 73 ]. Hypoxic conditions result in excessive cytosolic hyperacidification due to the accumulation of waste byproducts, including lactate, pyruvate, and acetic acid in the central zone [ 74 ]. Of course, the intensity of the pH value is blunted from the central zone to the outer layers. The activation of HIF-1α triggers the enzymes of the anaerobic glycolysis pathway and heightens the intracellular levels of lactate (Fig. 2 ) [ 70 , 75 , 76 ]. In the presence of HIF-1α, the function of the Na + /H + exchanger is altered to efflux accumulated proton ions and regulate intracellular pH homeostasis, leading to the reduction of ECM pH values [ 77 ]. Acidic conditions can increase the solubility of angiogenesis factors such as VEGF and activate relevant signaling pathway effectors such as MAPK/ERK in target cells. It should not be forgotten that excessive acidic conditions beyond the cell threshold can inhibit the angiogenic behaviors of ECs, leading to the promotion of necrotic cell death [ 78 , 79 ]. In support of this statement, Mena et al. found that acid preconditioning (pH = 6.6) of endothelial colony-forming cells promotes vasculogenesis, and increases the adhesion, and cell resistance in response to hyperglycemic conditions and pro-inflammatory status [ 61 ]. Of course, the intensity of the pH value is blunted from the central zone to the outer layers.

Copyright 2023. [ 79 ]. Cancer Cell International (Springer Nature Publishing Group). In the right panel, the schematic of tumor spheroid cell layers is indicated. Due to hyperacidification, lack of enough O 2 , and other parameters, most of the cells in the central zone undergo apoptotic and necrotic changes. In response to these features, juxtaposed to the central zone can release HIF-1α along with several angiogenesis factors to stimulate the formation of blood vessels. These vascular units are indicated by typical capillary sprouts in the tumor mass periphery and anastomosis within the stroma. The existence of hypoxic conditions is an essential element for the promotion of angiogenesis in tumor spheroids/tumoroids. The right panel was designed by using BioRender's web-based software
Typical tumor spheroid structure. In the left panel, the multicellular tumor spheroid is composed of human adenocarcinoma colorectal HT-29 cells, HUVECs, and HFFF2 fibroblasts. Spheroids have an inner dark compact core area and are enclosed by several cells in the periphery (outer layers). The connection of cells is loose in the external layers, indicating higher proliferation capacity.
In an experiment conducted by Taghavi Narmi et al., they developed colon cancer tumoroids using human HT-29 adenocarcinoma cells, HFFF2 fibroblasts, and umbilical cord vein ECs (HUVECs) using hanging drop embedded in 2.5% methylcellulose solution [ 79 ]. Based on histological analysis, central cells exhibit typical features of necrotic changes with prominent pyknotic changes [ 79 ]. These conditions promoted the production of angiogenesis factors, especially endocan in the outer layers [ 79 ]. Schmitz et al. indicated a significant reduction of O 2 levels in mesenchymal stem cell (MSC) spheroids using CPOx-orange polystyrene microbeads, and OPAL Optical O 2 measurement system by monitoring UnaG hypoxia reporter protein [ 80 ]. Data indicated O 2 levels dropped below 1% (v/v) in spheroids containing about 3 × 10 4 MSCs while these levels were near 2.5% (v/v) in a 2D culture system [ 80 , 81 ]. Of course, it should be kept in mind that local O 2 levels are variable in cell microaggregates developed using different fabrication platforms. For instance, the O 2 tension is higher in the deep layer of MSC spheroids induced by hanging drop methods using Terasaki plates when compared to spheroids fabricated by direct culture on ultra-low attachment plates [ 80 ]. The activation of vascularization and accumulation of metabolic byproducts can lead to differences in temperature values compared to the surrounding tissues [ 82 ]. It seems that the active pro-angiogenesis properties increase the local temperature within the solid tumor masses while the progression of necrotic changes, accumulation of metabolic byproducts, and reduction of metabolic activity reduce the local cancer mass temperature compared to the healthy niches [ 82 ]. In an interesting experiment conducted by Kumar et al., they proved a temperature difference between the 3D tumoroid core and periphery composed of human HCT-8 colon cancer cells and NIH3T3 fibroblast cells using the fluorescent polymeric nano-thermometers. Data indicated fewer temperature values (~ 2.9 °C) in the core zone compared to the outer layers of the tumoroid system [ 83 ]. Therefore, one can hypothesize that the active metabolic state along with higher temperature values can help us in the evaluation of pro-angiogenesis status within the tumor parenchyma in in vitro conditions. Hypothermic conditions in the core of tumor mass are highly related to the lack of angiogenesis signaling and prominent necrotic changes as seen in real tumor masses. Besides, these features can stimulate specific signaling molecular pathways. For instance, several signaling pathways related to inflammation, angiogenesis, and epithelial-mesenchymal transition (EMT) were indicated in hepatocellular organoids [ 84 ]. Of note, prolonged hypoxic conditions can result in the expression of angiogenesis-related factors such as VEGF within the organoid systems [ 85 ]. Regarding the fact that most tumor cell types prefer glycolysis over oxidative phosphorylation, it seems that the lack of suitable oxygen levels can educate these cells to behave similarly to the in vivo-like conditions. In organoids exposed to hypoxic conditions, the proliferation of ECs and differentiation of stem cells and progenitors toward endothelial lineage is increased [ 86 ]. Each cell type can select specific spatial localization within the organoid/tumoroid structure. For example, ECs prefer the periphery of spheroids/organoids in the early days after development while the formation of vascular units and elongation of these cells help them to penetrate the deeper layers (Fig. 3 ) [ 87 ]. In line with this statement, in heterotypic spheroids consisting of ECs and MSCs, green fluorescent ECs can be detected in the periphery of these structures over time and promotion of angiogenesis response increases the EC migration into the central zone according to gradient density of angiogenesis factors [ 87 ]. These features highlight the importance of EC location within the tumoroid/spheroid system which can pre-determines the angiogenesis capacity. It is postulated that the poor access of innermost cells of tumoroids to O 2 can initiate the pro-angiogenesis signaling pathways while new vessel formation is promoted by the stimulation of outer layer ECs. It was suggested that ECs in the periphery of unicellular spheroids are more potent in generating capillary sprouts and outgrowth within the collagen gel [ 65 ]. Inside the multicellular spheroids composed of human endothelial and osteoblast lineages, ECs can generate the CD31 + microvessels in in vitro conditions and these vascular units can interconnect with surrounding capillaries after being transplanted into the target tissues [ 88 ]. Using appropriate ECM components and suitable cell types, the angiogenic potential of vascular cells can be stimulated [ 89 ]. In spheroids composed of ECs and smooth muscle cells (SMCs) within the methacrylated hyaluronic acid with fibrinogen, the expression of angiogenesis factors such as stromal-derived factor-1 alpha, HIF-1α, and angiopoietin 1 and migration capacity were in the maximum levels in EC-SMC spheroids compared to EC, and SMC spheroids [ 89 ]. These data indicate that heterotypic interaction can promote the function of ECs and thereby angiogenesis potential inside the tumoroids/spheroids. In breast cancer tumoroids consisting of MDA-MB-231 and/or MCF-7 cancer cell lines, lung ECs, and bone marrow MSCs, the localization of ECs is associated with breast cancer cell types and close interaction with MSCs in tumoroids. Cellular organization was more prominent in tumoroids containing MDA-MB-231 rather than MCF-7 cells. This effect would be related to the supportive role of MSCs in the stabilization of vascular units via direct juxtacrine interaction with ECs or pericytes [ 90 , 91 ]. It is also mentioned that ECM-producing cells like fibroblasts can improve the angiogenic behavior of ECs within the tumoroid/spheroid structures [ 92 ]. Inside the body, fibroblasts, especially cancer-associated fibroblasts (CAFs), tend to localize in the core zone of tumors while proliferating cancer cells occupy the outer margins of solid tumors [ 93 ]. Of note, the exact location of fibroblasts within the tumoroid system determines morphological adaptation. For instance, CAFs and fibroblasts located near the core zone are round-shaped while these cells are elongated and flattened in the outer layer. This effect would be closely associated with topological net charge in which the deep layer exhibits positive values and these features are negative in the periphery of tumor masses [ 94 ]. It was shown that fibroblasts and especially CAFs can support tumor angiogenesis via the production of type I collagen, different pro-angiogenesis factors such as fibroblast growth factor (FGF), VEGF, etc., and stimulation of EMT phenomenon (Fig. 4 ) [ 95 , 96 ]. In contrast to the cancer cells, E-cadherin and other adhesion molecules are normally distributed at the surface of normal cells, increasing the juxtacrine cell-to-cell interaction over time [ 97 , 98 ]. Morphological changes and close interaction of cells within the spheroid/tumoroid system along with the hypoxic core are essential for angiogenesis potential [ 62 , 99 ]. Emerging data have revealed that there is a close relationship between spheroid/tumoroid vascularization potential and activation of resistance mechanisms [ 100 ]. Ahn and co-workers developed unicellular HepG2 cells, and hybrid HepG2 plus HUVEC spheroids using fibrinogen matrix [ 100 ]. Compared to unicellular HepG2 spheroids, the expression of genes related to angiogenesis and vascular unit function ( CD31 , vWF , and FLT1 ), and metastasis ( H19 , VIM , LAMB3 , ITGA5 ) were prominent in HepG2-HUVEC spheroids. These data confirmed that heterogeneous cell microstructures (such as tumoroids) can efficiently provide geometric clues for in vivo-like cell behavior and phenotype, especially cancer angiogenesis status [ 100 ]. Inside solid tumors, the existence of differentiation capacity from the epithelial-to-mesenchymal lineage, especially ECs, and other cell types can enhance the formation of intraparenchymal vessels, resulting in invasive tumor cell behavior and metastasis toward remote sites [ 101 ]. HepG2-HUVEC fibrin spheroids indicated eminent expression of EMT factors (LUG↑, MMP-9↑, and α-SMA↑), leading to the increase of intra-spheroid vascularization [ 100 ]. It has been indicated that ECs possess plasticity to acquire mesenchymal phenotype (EndMT) within the cancer parenchyma. Upon the activation of EndMT, ECs reduce protein levels of certain factors such as CD31, VE-cadherin, Tie-2, and vWF, and the expression of mesenchymal cell lineages such as α-SMA, type I/III collagen, and fibroblast-specific protein-1 is induced [ 102 ]. Inside the tumor niche, resistant cancer cells and CSCs prefer to locate at sites near the hypoxic zone to maintain their stemness features, angiogenesis potential (VEGF), and resistance mechanisms [ 103 ]. In contrast, the increase of O 2 levels inside TME leads to CSC-to-normal cancer cell maturation, and loss of stemness feature loss [ 104 ]. It should be noted that the development of vascular units within the tumoroid/spheroid system indicates activated endothelial differentiation of CSCs, and proliferation of ECs in response to hypoxic conditions. In the hypoxic conditions, glioma CSCs are prone to acquire EC phenotype by the activation of A3 adenosine receptor [ 105 ]. It was shown that the exposure of spheroids to specific culture conditions such as cyclic fluid shear stress can improve the angiogenesis potential and endothelial differentiation of adipose MSCs (vWF↑, and CD144↑) [ 106 ]. The application of specific ECM, and/or ECM-like substrates with different cell lineages (multicellular spheroids) can yield a suitable platform for the analysis of tumor angiogenesis. However, it should be kept in mind that providing a hypoxic niche inside spheroids/tumoroids using certain techniques is an essential factor in an efficient angiogenesis analysis. It is suggested that the mean diameter of avascular tumoroids/spheroids should exceed 400 µm for obtaining a hypoxic core [ 107 ].
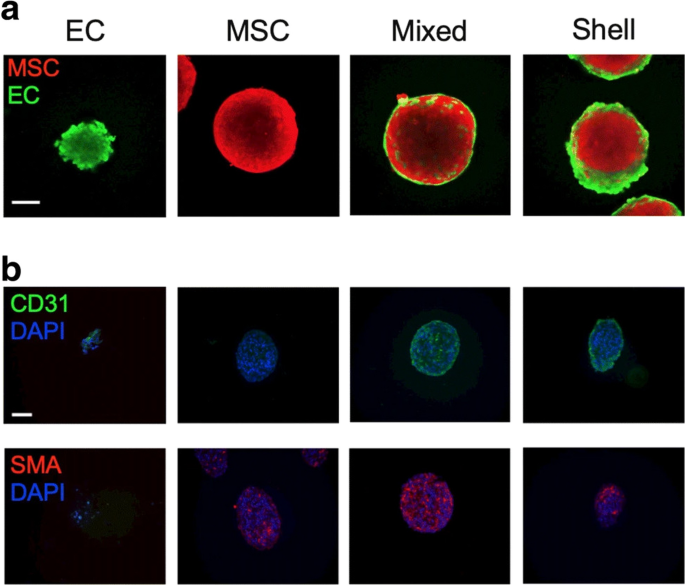
Copyright 2020 [ 228 ]. Journal of Molecular Medicine (Springer Nature Publishing Group)
The localization of human cord blood EC and bone marrow MSCs within the spheroids generated using agarose molds (Day 0 a – b ). ECs and MSCs were stained with CellTrace™ Oregon Green® 488, and CellTrace™ Far Red Cell, respectively to be tracked within the spheroid structure. Immunofluorescence images indicate the localization of ECs in the periphery of spheroids ( a , and b ). Data showed that green CD31 + cells were located at the periphery of spheroids while red-colored α-SMA. + MSCs distributed within the spheroid parenchyma. The nuclei were stained using DAPI. These data show that cell alignment and localization depend on types and functions within the spheroids in in vitro conditions.
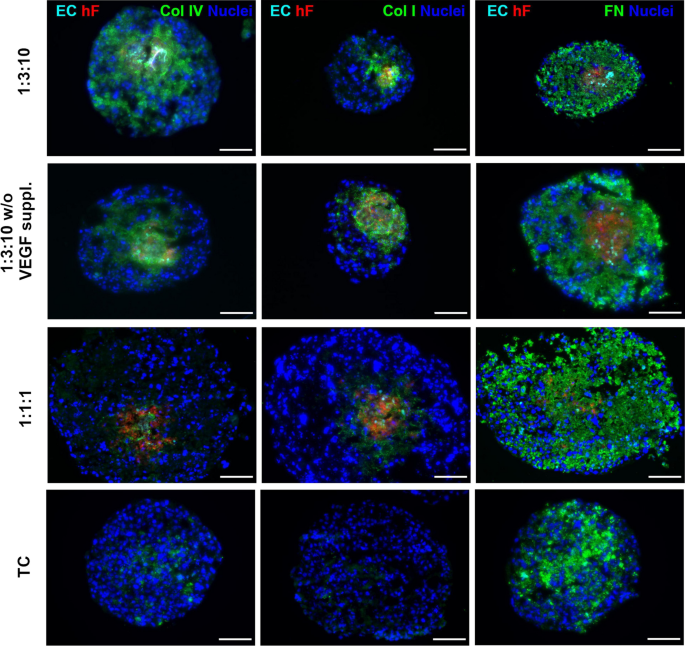
Copyright 2021. [ 236 ]. Frontiers in Bioengineering and Biotechnology (Frontiers Publishing Group)
Detection of ECM protein components [collagen IV (Col IV), collagen I (Col I), and fibronectin (FN)] in multicellular spheroids composed of breast HCC1954 cells (TC), PKH26-labeled dermal fibroblasts (hF), and Cell Tracker Deep Red-labeled HUVECs (ECs) with different ratios (TC 1: hF 1: EC 1, and TC 1: hF 3: EC 10) using immunofluorescence images. Spheroids were generated using ultra-low 96-well plates. Spheroids were incubated with VEGF [VEGF suppl. (0.5 ng/m)] or VEGF-deprived conditions (VEGF w/o) for 30 days, and exposed 2-day static culture system followed by agitation-based culture setting (rotation speed: 100 rpm). Data showed that fibronectin was produced in monotypic and heterotypic spheroid systems and is mainly at the center zone of spheroids, indicating the cancer cell origin. In contrast, the production of type I and IV collagen was promoted in the heterotypic spheroid system compared to the monotypic spheroids. The presence of VEGF can intensify the production of collagen by fibroblasts within the heterotypic spheroid system. These data highlight the role of cells in the production of certain components of ECM via the production of certain growth factors within the spheroid systems. The analyses were done in triplicate. Nuclei were stained with DAPI Scale bar, 100 μm.
During past decades, several techniques and assays have been used to assess the angiogenesis potential of cancer cell spheroids and tumoroids based on the EC function and activity. Among several techniques and approaches, spheroid-based sprouting angiogenesis is a simple, fast, and valid assay to measure the formation of new blood vessels and the activity of tip cells embedded in supporting dense matrices [ 67 ]. Sprouting parameters such as the number of sprouting and migrating ECs, mean envelope area, mean total outgrowth cell area, and the distance of ECs from the spheroid center, and border can be calculated in unicellular spheroids or multicellular spheroids composed of endothelial lineage and other cell types [ 108 , 109 , 110 ]. In an interesting study, Park and colleagues monitored the angiogenesis behavior of tumor spheroids composed of human fibroblasts with different cancer cell lines such as HepG2, U87MG, A549, and/or plasmacytoid dendritic cells in all-in-one-IMPACT system using the microfluidic platform and fibrin gel [ 111 ]. The formation of vascular sprouts within the different concentrations of fibrin hydrogel was assessed [ 111 ]. Several molecular analyses such as proteomic [ i.e . immunofluorescence [ 112 , 113 ], immunohistochemistry staining [ 114 ], western blotting [ 79 ], etc.], and genomic assays have been also to measure the angiogenesis status in several spheroid systems [ 62 , 79 , 115 ] following the exposure to different modalities [ 116 , 117 , 118 ].
Along with different in vitro spheroid-based angiogenesis assays, the transplantation of developed spheroids/tumoroids into animal models has been extensively used during the last decades [ 119 ]. Spheroid-plug model is one of the most applicable methods for the analysis of spheroid-derived angiogenesis in in vivo conditions. In this approach, unicellular and/or multicellular spheroids are embedded inside the supporting substrates, like Matrigel, etc., and directly injected into the subcutaneous area or transplanted into the target tissues [ 120 , 121 ]. At distinct time points, the angiogenesis potential of transplanted spheroids/tumoroids was assessed using imaging techniques, i.e . ultrasonographic, proteomic, and genomic analyses [ 122 ]. Recently, Choi and co-workers investigated the formation of angiogenesis in a mouse xenograft model of oral mucosa after the injection of CAFs and head and neck squamous cell carcinoma (SCC) grown in a 2D monolayer and/or 3D spheroid culture systems [ 123 ]. Histological analyses revealed that the number of CD31 + was less in mice that received disassociated cells while the injection of spheroids resulted in the formation of a vascular bed with numerous CD31 + cells [ 123 ]. Interestingly, they found that the levels of exosomes (Exos) also increased in spheroids composed of CAFs, and SCC cells with the increase of angiogenesis potential ( pdgf ↑, vegf ↑, vegfr-2 ↑, pdgfra ↑). Data indicated that the presence of CAFs can increase the angiogenic potential of FaDu squamous cell carcinoma [ 123 ]. It has been shown that Exos are valid theranostics for monitoring molecular signatures in the parent cells and loading certain cargo for therapeutic purposes [ 124 ]. Exos are produced directly by the activity of the endosomal system inside the cytosol via the invagination of the endosome membrane. During this process, several signaling factors and pro-angiogenesis factors are sequestrated into the Exo lumen [ 125 ].
Due to their nano-sized scales, Exos can easily penetrate the spheroid structure for the analysis of angiogenesis potential. In most studies, supporting ECM is composed of collagen, gelatin, hyaluronic acid, polymeric carbohydrates such as alginate, and other substrates which can yield fibrous networks or hydrogel with nano- to micron-sized pores in the structure of spheroids/tumoroids [ 126 , 127 , 128 ]. To this end, Capik and co-workers incubated human EC spheroids with hypoxic oral SCC cell Exos for monitoring the angiogenesis potential [ 129 ]. Data indicated that exosomal miR-1825 led to the increase of sprouts in EC spheroids via the modulation of the TSC2/mTOR axis [ 129 ]. These data confirmed that Exos can efficiently harbor both genetics and proteomics in different cell layers within the spheroid structure. Whether the majority of Exos can be uptaken by cells located at outer or deep cell layers needs more elucidation. In an interesting study, paclitaxel/gemcitabine monophosphate-loaded Exos efficiently entered pancreatic ductal adenocarcinoma spheroids with about an average diameter of 300–350 µm. Immunofluorescence images revealed significant homing capability of fluorochrome-stained Exos in the deep layer of pancreatic ductal adenocarcinoma spheroids and orthotopic model in mice [ 130 ]. Hao and co-workers indicated the prominent penetrating properties of doxorubicin-loaded Exos in human adenocarcinoma A549 spheroids after 4 h in in vitro conditions [ 131 ]. These data show that Exos are valid diagnostics for the evaluation of pro-angiogenesis cargo. Thus, Exos can be used for the delivery of specific therapeutics into deep layers of tumoroids/spheroids using some sophisticated loading techniques.
Microfluidic devices and 3D bioprinting for fabrication of vascularized tumor spheroids
In recent years, 3D bioprinting technology along with microfluidic devices have been used for precise and spatial arrangement of cells and supporting materials to generate micro-sized aggregates and simulate in vivo tumor-like conditions [ 132 ]. Along with different bioprinting modalities, droplet-based bioprinting (such as inkjet, acoustic, and microvalve-based approaches) exhibits reproducibility, accuracy, adaptability to different substrates, etc. for the fabrication of tumor spheroids/organoids [ 133 ]. Using droplet-based bioprinting, both cell number and type can be controlled in the structure of final tumor spheroids [ 133 ]. Previously, Utama et al. used drop-on-demand bioprinter and alginate-based substrate for the fabrication of multicellular tumor spheroids (neuroblastoma, and lung cancer cell lines) with the potential to control parameters such as size, and dimensions of colonies [ 134 ]. Data indicated a rapid spheroid formation rate with high encapsulated cell numbers within certain dimensions. The current approach was eligible to preserve the stemness of neuroblastoma cells (CD133 + cells↑) within the tumor spheroids. Besides, the cell alignment and compactness were increased in 3D-bioprinted spheroids as compared with manually prepared spheroid counterparts. The presence of HIF-1α + cells indicates typical hypoxic conditions in the inner layers of 3D-bioprinted spheroids which can be used for monitoring angiogenesis and drug screening [ 134 ]. In a similar work conducted by Hong and co-workers, they used a 3D bioprinting system for the evaluation of drug resistance in breast cancer spheroids containing MCF-7 CSCs and alginate-gelatin hydrogel [ 135 ]. The developed tumor spheroids preserve the stemness feature (CD44 + /CD24 − /ALDH + cells↑) with simultaneous up-regulation of resistance markers such as GRP78 chaperon and ABCG2 [ 135 ].
In recent years microfluidic bioreactors have been increasingly used for the evaluation of angiogenesis response in tumor spheroids [ 100 ]. It has been shown that the integration of the tumor-on-a-chip technique with 3D bioprinting platforms can help us provide a heterogeneous TME and monitor angiogenesis as well [ 136 ]. Meng and co-workers fabricated 3D bioprinted modules consisting of photo-responsive microcapsules [GelMA core with EGF, and Au-functionalized PLGA film], A549 tumor cell droplets, endothelialized (HUVECs) microchannels, and fibroblast-loaded natural hydrogel. This platform can successfully mimic the TME and be eligible to monitor cancer cell migration in association with growth factor gradient and interaction with the stromal cells [ 137 ]. By combining 3D bioprinting technology and microfluidic devices, unicellular or multicellular tumor spheroids can be exposed directly to an EC-lined surface with predetermined flow rates and certain pro-, and anti-angiogenesis factors [ 138 ]. The angiogenesis rate is monitored in terms of vascularization area, and penetration of vascular cells to spheroid structure (Figs. 5 , and 6 ) [ 100 ]. Han and co-workers studied the angiogenesis of U78 glioblastoma tumoroids plated on a bioprinted vascular niche composed of pulmonary fibroblasts and human ECs embedded in gelatin/alginate/fibrinogen composite [ 139 ]. Data confirmed the invagination of vascular ECs into tumoroid structure and migration of U78 cancer cells toward vessels and vice versa. On day 7, the expression of CD31 and α-actin is up-regulated coinciding with the formation of vascular network within the hydrogel. In the presence of anti-cancer compounds (temozolomide, and sunitinib), the intensity of vascular units was reduced in the tumoroid backbone compared to the cells treated with temozolomide alone. These features indicate the feasibility of the designed system for monitoring the efficiency of several anti-cancer drugs on certain tumor types in vitro [ 139 ].
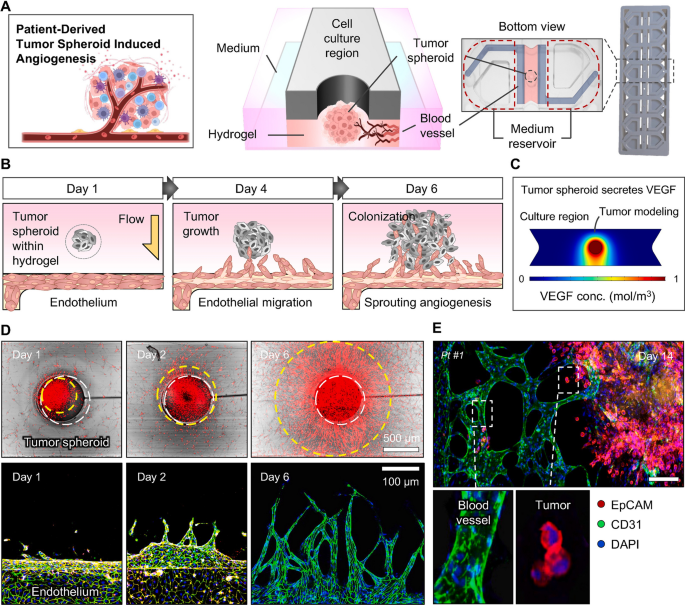
Copyright 2024. [ 138 ]. Biomaterials (Elsevier Publishing Group)
Application of microfluidic 3D culture system for monitoring the patient tumor (gastric adenocarcinoma)-derived spheroid angiogenesis response ( A – E ). The patient-derived tumor spheroids were generated using fibrin hydrogel. The microfluidic 3D culture system provided a valuable platform to measure the angiogenesis properties of spheroids after being exposed to HUVECs in the EC channel ( A ). The reciprocal interaction between the EC layer and hydrogel-embedded spheroids within the microfluidic 3D culture system ( B ). The existence of hydrostatic pressure stimulated tumor spheroids to release angiogenesis-related factors such as VEGF toward the EC channel ( C ). Tumor cell and EC growth were monitored using confocal microscopy for 6 days ( D ). After 14 days, the fluorescein-labeled Ulex Europaeus agglutinin I + EC layer advanced toward Alexa Fluor 594-EpCAM. + tumor spheroid to generate vascularized tumor mass. Scala bar: 10 µm.

Copyright 2023. [ 100 ]. Acta Biomaterialia (Elsevier Publishing Group)
Comparison of lymphangiogenesis efficiency in tumor spheroids inside microfluidic chip device exposed to static and dynamic culture condition ( a-e ). Schematic illustration of interstitial flow effect on lymphangiogenesis and angiogenesis properties of tumor spheroids composed of fibrin-embedded HepG2 cells and HUVECs. Tumor spheroids (HepG2 cells + HUVECs) were injected and fixed in the central channel using fibrin clots containing vascular ECs and lymphatic ECs ( a ). Confocal images of angiogenesis (red-colored Alexa Fluor® 594 CD31 + cells) and lymphangiogenesis (green-colored Alexa Fluor® 488 podoplanin. + cells) under static and dynamic culture conditions ( b ) Scale bars: 400 μm). Spheroid size ( c ), vascularization area ( d ), and lymphatic vessel area ( e ) were measured in conditions with static and dynamic culture systems (n = 4). Data indicated that both lymphangiogenesis and angiogenesis were stimulated in tumor spheroid systems after being exposed to a dynamic culture system. Non-paired student's t-test. *** p < 0.001. n.s: non-significant.
Under physiological conditions, the existence of shear stress with frictional forces is an essential element for the regulation of EC function and activity. Thus, the application of a perfusable culture system with simultaneous shear stress forces can yield reliable data compared to static culture platforms. In microfluidic systems, microfabrication approaches can be used for the fabrication of microchannels using suitable substrates for monitoring cell attachment, morphogenesis, and function in the dynamic aqueous phase [ 140 ]. In an interesting work, Miller and co-workers developed engineered vascular tissue consisting filament network composed of carbohydrate glass using 3D bioprinting [ 141 ]. The luminal surface was furnished with ECs and exposed to pulsative blood flow. Based on the complexity of TME, this approach can be applied to control several geometries such as vascular network dimension, EC function, impact of intervascular niche, and tumor cell behavior. The application of different cell lineages within certain substrates is also possible for achieving reliable in vivo-like data [ 141 ]. Using a tumor microfluidic model, immune cell function can be also monitored in response to tumoroids with different cell types [ 142 ]. In this regard, fibroblasts were co-incubated with different tumor cells (H69M lung cancer cells, OV90 ovarian cancer cells. SN12C kidney cancer cells) within the tumor-on-a-chips composed of PDMS for assessing angiogenesis potential [ 142 ]. The tumoroids were overlaid on HUVEC- and fibroblast-incorporated fibrin gel and vascularized tumoroids were evident after 7 days [ 142 ]. Application of continuous flow for 4 days showed that CAR T cells successfully penetrate the tumoroid microenvironment and interact with the apical surface vascular ECs. Along with the recruitment of CAR T cells into the tumor niche, the local IFN-γ levels were also increased, indicating the activation of CAR T cells after being exposed to tumor cells [ 142 ].
In most previously conducted works, ECs have been used as the main vascular cell component for monitoring angiogenesis in tumor model analyses. The inclusion of other vascular cells such as pericytes and perivascular niches can reflect more reliable in vitro data about the dynamic tumor cell growth comparably to in vivo conditions [ 143 ]. In an experiment conducted by Ngo et al., brain glioblastoma tumoroids were fabricated using brain microvascular ECs, pericytes, and astrocytes to monitor the possible role of perivascular cells in angiogenesis potential of tumoroids within the GelMA substrate [ 143 ]. Data indicated that mono, di, and triculture of ECs with pericytes, and astrocytes led to the formation of a primitive stable vascular network (CD31↑, ZO↑) within the tumor unicellular or multicellular aggregates. The presence of pericytes can increase the expression of tight junction molecules such as ZO, and CLDN5. Along with these changes, the expression of ECM components such as LAMA4 was also induced. The culture of GBM6 cell spheroids within the GelMA hydrogel containing ECs, pericytes, and astrocytes indicated numerous peripheral invading tumor cells after 7 days. On the other hand, ECs were also recruited to the proximity of tumor spheroids to generate vascular networks. Taken together, the existence of perivascular cells (pericytes) can stimulate the migration and metastasis of glioblastoma cells with enhanced vascular density [ 143 ].
Current bottlenecks and challenges
Despite the superiority of unicellular and multicellular spheroids over 2D culture systems in terms of cancer research, this platform faces some critical limitations that need further consideration. Compared to cultured cells in 2D systems, bright-field imaging and obtaining high-resolution scanning are usually difficult and laborious due to intricate geometries that affect light scattering, penetration, and absorption within the spheroid/tumoroid systems [ 144 , 145 ]. These features make it difficult to make transparent images and study the central zone of multicellular complexes. Prolonged light exposure can also increase the possibly of phototoxicity and photobleaching effects that per se necessitate the application of more expensive techniques such as electron microscopy, and layer-by-layer fluorescence imaging [ 146 , 147 ]. In most circumstances, the lack of an appropriate penetration rate or homogenous distribution of fluorochrome compounds reduces the possibility of certain biomarkers and positive cells within the deep layers of spheroid/tumoroid systems [ 7 , 144 , 148 ]. To fabricate interpretable 3D datasets, certain imaging software, and platforms with motorized and controllable microscope frames are mandatory [ 144 ]. It should not be forgotten that the mean diameter size of multicellular units is not completely similar which can affect the access of nutrients and other essential factors into central zones. In this regard, obtaining almost uniform data is problematic and makes the results difficult [ 149 ]. In contrast to in vivo conditions, the distribution of O 2 and various nutrients in the 3D culture system is restricted in the static culture systems. Of note, dynamic culture platforms can in part circumvent these pitfalls but it is not completely similar to biological systems [ 150 ]. In terms of anatomical microstructure, the tumor masses are enclosed by solid tissues while in in vitro spheroid/tumoroid cultures are surrounded by aqueous phases. These features allow the cells located at the external surface to proliferate more actively because of unlimited access to growth factors and cell culture elements [ 7 ].
Notwithstanding, each tumor has specific supporting ECM components and the development of spheroid/tumoroid systems with native ECM compositions needs standard protocols and sophisticated synthesis protocols. It is believed that ECM composition and variation in supporting substrates can affect the genetic traits, cellular behavior within the spheroid/tumoroid complex, and response to therapeutics and drug screening protocols [ 144 , 151 ]. Compared to the real tumor mass, the in vitro multicellular systems possess a relatively simple microstructure with a limited number of ECM components. The entity of ECM can change during the culture periods and selection of cell ratio in tumoroid systems. Therefore, the selection of appropriate cells with relatively similar tumor mass ratios is mandatory to establish more realistic models and compare the in vitro and in vivo data [ 150 ]. In most spheroid/tumoroid models, the lack of microstructure interconnectivity, ECM alignment, and specific cell types such as cancer-associated fibroblasts (CAFs), tumor-associated macrophages (TAMs), and other vascular components such as pericytes can affect the dynamic growth of cancer cells and tumor mass behavior [ 144 , 152 ]. Unlike in vivo conditions, spheroids/tumoroids are devoid of neuroimmunoendocrine interactions which allows cancer cells to proliferate more actively compared to other cell types [ 150 ]. It is believed that M2 TAMs can exert immunosuppressive properties with the potential to change tumor cell migration, drug sensitivity and ECM components [ 153 ]. Whether and how co-cultured fibroblasts can acquire in vivo-like stable phenotypes (CAFs) is associated with the production of several cytokines, and physical interaction with the other cells [ 150 ]. CAFs are actively present in the tumor matrix and likely produce a large fraction of ECM components (fibronectin, collagen, etc.) along with certain growth factors. It has been thought the number of CAFs and ECM production correlate with stable and continuous hypoxic conditions and low pH values [ 153 ]. The co-culture of ECs with other cells within the multicellular system cannot fully mimic tumor parenchyma vascular networks while constant shear stress and perfusion should be provided [ 154 ]. Direct evidence for low-content intercellular junction factors, i.e., E-cadherin, leads to formation of less compact spheroid/tumoroid system. In most circumstances, the up-regulation of N-cadherin instead of E-cadherin loosen the cell-to-cell interaction [ 146 ]. Of course, certain ECM components such as collagen can facilitate the compactness of spheroids/tumoroids. Unfortunately, creating a multicellular system with a dense collagen matrix is somehow impossible and needs intricate synthesis protocols that may damage the cells [ 145 , 146 ]. These features closely affect the penetration and tumoricidal properties of immune cells [ 150 , 155 ].
Conclusions
Tumoroids/spheroids provide a valid cost-effective, fast, precise, and reproducible in vitro platform to recapitulate in vivo-like conditions for the analysis of angiogenesis status in response to drugs and other factors [ 156 ]. Besides, both multicellular and unicellular tumor spheroids enable us to study the paracrine and juxtacrine interaction between the homotypic and heterotypic cells. In most previously conducted experiments, several parameters such as tumor cell dynamic, metastasis, drug screening, etc. have been analyzed more than the angiogenesis potential. Thus, the current review article aims to scrutinize the importance of angiogenesis/vascularization in the dynamic growth of the tumoroid/spheroid system. Based on several studies, endothelial lineage, and/or stem cell sources are used to mimic blood vessel structure within the multicellular and unicellular tumor spheroids, and thus type, intensity, and duration of angiogenesis responses can differ based on the cell components and supporting matrices. Like tumoroids composed of different cell lines, patient-derived tumoroids are also valid analytic tools to find appropriate medications and screen novel therapies targeting the angiogenesis potential. It should not be forgotten that tumoroids/spheroids have their limitations despite several advantages compared to conventional 2D culture systems. The entity, structure, and vascular cells involved in the development of vascular niches are not completely similar to in vivo conditions. Thus, additional efforts are needed to recapitulate different aspects of the tumor microenvironment, such as the inclusion of immune cells recapitulation of appropriate vessel morphologies, or appropriate basement membrane or ECM composition. Finding the exact in vivo-like cell-to-cell ratio is another challenge that restricts the applicability of 3D culture system-based assays for therapeutic purposes. Commensurate with these facts, future studies are suggested to address these issues for the development of powerful culture system tools comparable to in vivo tumor conditions.
Availability of data and materials
Data sharing is not possible as no new data were created or analyzed in this study.
Abbreviations
Cancer stem cells
Cancer-associated fibroblasts
Endothelial cells
Endothelial progenitor cells
Endothelial-mesenchymal transition
Epithelial-mesenchymal transition
Extracellular matrix
Fibroblast growth factor
Hypoxia-inducible factor 1 alpha
Mesenchymal stem cells
Poly (lactic-co-glycolic acid)
Polyethylene glycol
Polyurethane
Smooth muscle cells
Squamous cell carcinoma
Three dimension
Transforming growth factor-β
- Tumor microenvironment
Tumor-associated macrophages
Two dimension
Umbilical cord vein ECs
Vascular endothelial growth factor
Zhao Z, et al. Organoids. Nat Rev Methods Primers. 2022;2(1):94.
Article PubMed PubMed Central CAS Google Scholar
Cacciamali A, Villa R, Dotti S. 3D cell cultures: evolution of an ancient tool for new applications. Front Physiol. 2022;13: 836480.
Article PubMed PubMed Central Google Scholar
Yin S, et al. Composite microfluidic petri dish-chip (MPD-Chip) without protein coating for 2D cell culture. Langmuir. 2023;39(44):15643–52.
Article PubMed CAS Google Scholar
Lerman MJ, et al. The evolution of polystyrene as a cell culture material. Tissue Eng Part B Rev. 2018;24(5):359–72.
Gao W, et al. Development of a novel and economical agar-based non-adherent three-dimensional culture method for enrichment of cancer stem-like cells. Stem Cell Res Ther. 2018;9(1):243.
Shah DD, et al. Harnessing three-dimensional (3D) cell culture models for pulmonary infections: state of the art and future directions. Naunyn Schmiedebergs Arch Pharmacol. 2023;396(11):2861–80.
Jubelin C, et al. Three-dimensional in vitro culture models in oncology research. Cell Biosci. 2022;12(1):155.
Law AMK, et al. Advancements in 3D cell culture systems for personalizing anti-cancer therapies. Front Oncol. 2021;11:782766.
Wang L, et al. Spatial topology of organelle is a new breast cancer cell classifier. iScience. 2023;26(7):107229.
Venkatesan M, et al. Spatial subcellular organelle networks in single cells. Sci Rep. 2023;13(1):5374.
Russell S, et al. Metabolic profiling of healthy and cancerous tissues in 2D and 3D. Sci Rep. 2017;7(1):15285.
Duval K, et al. Modeling physiological events in 2D vs. 3D cell culture. Physiology (Bethesda). 2017;32(4):266–77.
PubMed CAS Google Scholar
Dravid A, et al. A macroscopic diffusion-based gradient generator to establish concentration gradients of soluble molecules within hydrogel scaffolds for cell culture. Front Chem. 2019;7:638.
Montagner M, Dupont S. Mechanical forces as determinants of disseminated metastatic cell fate. Cells. 2020. https://doi.org/10.3390/cells9010250 .
Baker BM, Chen CS. Deconstructing the third dimension–how 3D culture microenvironments alter cellular cues. J Cell Sci. 2012;125(13):3015–24.
PubMed PubMed Central CAS Google Scholar
Saraswathibhatla A, Indana D, Chaudhuri O. Cell–extracellular matrix mechanotransduction in 3D. Nat Rev Molecular Cell Biol. 2023;24:1–22.
Article Google Scholar
Jensen C, Teng Y. Is it time to start transitioning from 2D to 3D cell culture? Front Mol Biosci. 2020;7:33.
Habanjar O, et al. 3D cell culture systems: tumor application, advantages, and disadvantages. Int J Mol Sci. 2021;22(22):12200.
Petersen OW, et al. Interaction with basement membrane serves to rapidly distinguish growth and differentiation pattern of normal and malignant human breast epithelial cells. Proc Natl Acad Sci. 1992;89(19):9064–8.
Sun M, et al. 3D cell culture—can it be as popular as 2d cell culture? Adv Nanobiomed Res. 2021;1(5):2000066.
Article CAS Google Scholar
Redmond J, et al. Advances in biofabrication techniques for collagen-based 3D in vitro culture models for breast cancer research. Mater Sci Eng C. 2021;122: 111944.
Nyga A, Cheema U, Loizidou M. 3D tumour models: novel in vitro approaches to cancer studies. J Cell Commun Signaling. 2011;5:239–48.
Moscona A, Moscona H. The dissociation and aggregation of cells from organ rudiments of the early chick embryo. J Anat. 1952;86(Pt 3):287.
Peng K, et al. HIF-1α promotes kidney organoid vascularization and applications in disease modeling. Stem Cell Res Ther. 2023;14(1):336.
Li X, et al. Assay establishment and validation of a high-throughput organoid-based drug screening platform. Stem Cell Res Ther. 2022;13(1):219.
Cacciamali A, Villa R, Dotti S. 3D cell cultures: evolution of an ancient tool for new applications. Front Physiol. 2022;13:836480.
Akhtar A. The flaws and human harms of animal experimentation. Camb Q Healthc Ethics. 2015;24(4):407–19.
Van Norman GA. Limitations of animal studies for predicting toxicity in clinical trials: is it time to rethink our current approach? JACC Basic Trans Sci. 2019;4(7):845–54.
Google Scholar
Florian S, et al. A human organoid system that self-organizes to recapitulate growth and differentiation of a benign mammary tumor. Proc Natl Acad Sci USA. 2019;116(23):11444–53.
Lee S-Y, et al. In Vitro three-dimensional (3D) cell culture tools for spheroid and organoid models. SLAS Discovery. 2023;28(4):119–37.
Xu H, et al. Tumor organoids: applications in cancer modeling and potentials in precision medicine. J Hematol Oncol. 2022;15(1):58.
Tatla AS, et al. A vascularized tumoroid model for human glioblastoma angiogenesis. Sci Rep. 2021;11(1):19550.
Gunti S, et al. Organoid and spheroid tumor models: techniques and applications. Cancers (Basel). 2021;13(4):874.
Zhou Z, et al. Evaluation of the tumoricidal efficacy of adoptive cell transfer using hepatocellular carcinoma-derived organoids. J Gastrointest Oncol. 2022;13(2):732–43.
Makouei F, et al. 3D ultrasound versus computed tomography for tumor volume measurement compared to gross pathology-a pilot study on an animal model. J Imaging. 2022;8(12):329.
Pasini A, et al. Perfusion flow enhances viability and migratory phenotype in 3D-cultured breast cancer cells. Ann Biomed Eng. 2021;49(9):2103–13.
Atat OE, et al. 3D modeling in cancer studies. Hum Cell. 2022;35(1):23–36.
Li W, et al. 3D Biomimetic Models to Reconstitute Tumor Microenvironment In Vitro: spheroids, Organoids, and Tumor-on-a-Chip. Adv Healthcare Mater. 2023;12(18):2202609.
Kang SM, et al. Engineered microsystems for spheroid and organoid studies. Adv Healthc Mater. 2021;10(2):2001284.
Wang Y, Sun Y. Engineered organoids in oral and maxillofacial regeneration. iScience. 2023;26(1):105757.
Wang D, et al. Hyaluronic acid methacrylate/pancreatic extracellular matrix as a potential 3D printing bioink for constructing islet organoids. Acta Biomater. 2023;165:86–101.
Heo JH, et al. Engineering the extracellular matrix for organoid culture. Int J Stem Cells. 2022;15(1):60–9.
Liu H, et al. Advances in hydrogels in organoids and organs-on-a-chip. Adv Mater. 2019;31(50):1902042.
Cheng Y, et al. Sustained hedgehog signaling in medulloblastoma tumoroids is attributed to stromal astrocytes and astrocyte-derived extracellular matrix. Lab Invest. 2020;100(9):1208–22.
Cesarz Z, Tamama K. Spheroid culture of mesenchymal stem cells. Stem Cells Int. 2016;2016(1):9176357.
Article PubMed Google Scholar
Lefort CT, Wojciechowski K, Hocking DC. N-cadherin cell-cell adhesion complexes are regulated by fibronectin matrix assembly*. J Biol Chem. 2011;286(4):3149–60.
Maître JL, Heisenberg CP. Three functions of cadherins in cell adhesion. Curr Biol. 2013;23(14):R626–33.
Dash S, et al. Heterophilic recognition between E-cadherin and N-cadherin relies on same canonical binding interface as required for E-cadherin homodimerization. Arch Biochem Biophys. 2022;727: 109329.
Wu SK, Yap AS. Patterns in space: coordinating adhesion and actomyosin contractility at E-cadherin junctions. Cell Commun Adhes. 2013;20(6):201–12.
Powan P, et al. Detachment-induced E-cadherin expression promotes 3D tumor spheroid formation but inhibits tumor formation and metastasis of lung cancer cells. Am J Physiol Cell Physiol. 2017;313(5):C556–66.
Zisis T, et al. Disentangling cadherin-mediated cell-cell interactions in collective cancer cell migration. Biophys J. 2022;121(1):44–60.
Derycke LD, Bracke ME. N-cadherin in the spotlight of cell-cell adhesion, differentiation, embryogenesis, invasion and signalling. Int J Dev Biol. 2004;48(5–6):463–76.
Ardizzone A, et al. Role of basic fibroblast growth factor in cancer: biological activity, targeted therapies, and prognostic value. Cells. 2023;12(7):1002.
Jia T, et al. FGF-2 promotes angiogenesis through a SRSF1/SRSF3/SRPK1-dependent axis that controls VEGFR1 splicing in endothelial cells. BMC Biol. 2021;19:1–26.
Yuan Y, et al. Role of the tumor microenvironment in tumor progression and the clinical applications (Review). Oncol Rep. 2016;35(5):2499–515.
Xu S, et al. The role of collagen in cancer: from bench to bedside. J Transl Med. 2019;17:1–22.
Borst R, Meyaard L, Pascoal Ramos MI. Understanding the matrix: collagen modifications in tumors and their implications for immunotherapy. J Trans Med. 2024;22(1):382.
Nazari SS. Generation of 3D tumor spheroids with encapsulating basement membranes for invasion studies. Curr Protoc Cell Biol. 2020;87(1): e105.
Namjoo AR, et al. Tissue engineering modalities in skeletal muscles: focus on angiogenesis and immunomodulation properties. Stem Cell Res Ther. 2023;14(1):90.
Chen K, et al. Comprehensive insight into endothelial progenitor cell-derived extracellular vesicles as a promising candidate for disease treatment. Stem Cell Res Ther. 2022;13(1):238.
Narmi MT, et al. Melatonin blunted the angiogenic activity in 3D colon cancer tumoroids by the reduction of endocan. Cancer Cell Int. 2023;23(1):118.
Beloglazova I, et al. New insight on 2D in vitro angiogenesis models: all that stretches is not a tube. Cells. 2022. https://doi.org/10.3390/cells11203278 .
Kapałczyńska M, et al. 2D and 3D cell cultures—a comparison of different types of cancer cell cultures. Arch Med Sci. 2018;14(4):910–9.
PubMed Google Scholar
Liu X, Bouman Chen Z. How their environment influences endothelial cells. Elife. 2023;12:e88248.
Heiss M, et al. Endothelial cell spheroids as a versatile tool to study angiogenesis in vitro. FASEB J. 2015;29(7):3076–84.
Liu Z-L, et al. Angiogenic signaling pathways and anti-angiogenic therapy for cancer. Signal Transduct Target Ther. 2023;8(1):198.
Kannan P, Schain M, Lane DP. An automated quantification tool for angiogenic sprouting from endothelial spheroids. Front Pharmacol. 2022;13:883083.
Guan Y, et al. Effects of hypoxia on cerebral microvascular angiogenesis: benefits or damages? Aging Dis. 2023;14(2):370.
PubMed PubMed Central Google Scholar
Raghavan S, et al. Comparative analysis of tumor spheroid generation techniques for differential in vitro drug toxicity. Oncotarget. 2016;7(13):16948–61.
Suarez-Martinez E, et al. 3D and organoid culture in research: physiology, hereditary genetic diseases and cancer. Cell Biosci. 2022;12(1):39.
Wicks EE, Semenza GL. Hypoxia-inducible factors: cancer progression and clinical translation. J Clin Invest. 2022. https://doi.org/10.1172/JCI159839 .
Yuan X, et al. Targeting hypoxia-inducible factors: therapeutic opportunities and challenges. Nat Rev Drug Discovery. 2024;23(3):175–200.
Magar AG, et al. A molecular perspective on HIF-1α and angiogenic stimulator networks and their role in solid tumors: an update. Int J Mol Sci. 2024;25(6):3313.
Pang X, et al. Weak acids produced during anaerobic respiration suppress both photosynthesis and aerobic respiration. Nat Commun. 2023;14(1):4207.
Pinto B, et al. Three-dimensional spheroids as in vitro preclinical models for cancer research. Pharmaceutics. 2020;12(12):1186.
Zhu Y, et al. 3D tumor spheroid and organoid to model tumor microenvironment for cancer immunotherapy. Organoids. 2022;1(2):149–67.
Kreitzer MA, et al. ATP-mediated increase in H+ efflux from retinal Müller cells of the axolotl. J Neurophysiol. 2024;131(1):124–36.
Faes S, et al. Acidic pH reduces VEGF-mediated endothelial cell responses by downregulation of VEGFR-2 relevance for anti-angiogenic therapies. Oncotarget. 2016;7(52):86026.
Mena HA, et al. Acidic preconditioning of endothelial colony-forming cells (ECFC) promote vasculogenesis under proinflammatory and high glucose conditions in vitro and in vivo. Stem Cell Res Ther. 2018;9(1):120.
Schmitz C, et al. Hypoxia onset in mesenchymal stem cell spheroids: monitoring with hypoxia reporter cells. Front Bioeng Biotechnol. 2021;9: 611837.
Schmitz C, et al. Live reporting for hypoxia: hypoxia sensor–modified mesenchymal stem cells as in vitro reporters. Biotechnol Bioeng. 2020;117(11):3265–76.
Knapp JP, et al. Tumor temperature: friend or foe of virus-based cancer immunotherapy. Biomedicines. 2022;10(8):2024.
Kumar A, et al. Synthesis and characterization of a fluorescent polymeric nano-thermometer: dynamic monitoring of 3D temperature distribution in co-cultured tumor spheroids. Analyst. 2023;148(9):2045–57.
Wang Y, et al. Microenvironment of a tumor-organoid system enhances hepatocellular carcinoma malignancy-related hallmarks. Organogenesis. 2017;13(3):83–94.
Schumacher A, et al. Enhanced microvasculature formation and patterning in iPSC–derived kidney organoids cultured in physiological hypoxia. Front Bioeng Biotechnol. 2022;10: 860138.
Podkalicka P, et al. Hypoxia as a driving force of pluripotent stem cell reprogramming and differentiation to endothelial cells. Biomolecules. 2020;10(12):1614.
Vorwald CE, Joshee S, Leach JK. Spatial localization of endothelial cells in heterotypic spheroids influences Notch signaling. J Mol Med (Berl). 2020;98(3):425–35.
Walser R, et al. Generation of co-culture spheroids as vascularisation units for bone tissue engineering. Eur Cell Mater. 2013;26:222–33.
Zuo X, et al. Spheroids of endothelial cells and vascular smooth muscle cells promote cell migration in hyaluronic acid and fibrinogen composite hydrogels. Research. 2020. https://doi.org/10.34133/2020/8970480 .
Rajan AM, et al. Dual function of perivascular fibroblasts in vascular stabilization in zebrafish. PLoS Genet. 2020;16(10): e1008800.
Nassiri SM, Rahbarghazi R. Interactions of mesenchymal stem cells with endothelial cells. Stem Cells Dev. 2013;23(4):319–32.
Lamichhane SP, et al. Recapitulating epithelial tumor microenvironment in vitro using three dimensional tri-culture of human epithelial, endothelial, and mesenchymal cells. BMC Cancer. 2016;16(1):1–12.
Sarkar M, et al. Cancer-associated fibroblasts: the chief architect in the tumor microenvironment. Front Cell Dev Biol. 2023;11:1089068.
Turiv T, et al. Topology control of human fibroblast cells monolayer by liquid crystal elastomer. Sci Adv. 2020;6(20):eaaz6485.
Hughes CC. Endothelial-stromal interactions in angiogenesis. Curr Opin Hematol. 2008;15(3):204.
Huang B, Huang M, Li Q. Cancer-associated fibroblasts promote angiogenesis of hepatocellular carcinoma by VEGF-mediated EZH2/VASH1 pathway. Technol Cancer Res Treat. 2019;18:1533033819879905.
Lin RZ, et al. Dynamic analysis of hepatoma spheroid formation: roles of E-cadherin and beta1-integrin. Cell Tissue Res. 2006;324(3):411–22.
Steinberg MS. Differential adhesion in morphogenesis: a modern view. Curr Opin Genet Dev. 2007;17(4):281–6.
Zhou Y, et al. 3D culture increases pluripotent gene expression in mesenchymal stem cells through relaxation of cytoskeleton tension. J Cell Mol Med. 2017;21(6):1073–84.
Ahn J, et al. 3D microengineered vascularized tumor spheroids for drug delivery and efficacy testing. Acta Biomater. 2023;165:153–67.
Yoshimatsu Y, Watabe T. Emerging roles of inflammation-mediated endothelial–mesenchymal transition in health and disease. Inflammation Regeneration. 2022;42(1):9.
Choi KJ, et al. Endothelial-to-mesenchymal transition in anticancer therapy and normal tissue damage. Exp Mol Med. 2020;52(5):781–92.
Emami Nejad A, et al. The role of hypoxia in the tumor microenvironment and development of cancer stem cell: a novel approach to developing treatment. Cancer Cell Int. 2021;21(1):62.
Kim H, et al. The hypoxic tumor microenvironment in vivo selects the cancer stem cell fate of breast cancer cells. Breast Cancer Res. 2018;20:1–15.
Rocha R, et al. The adenosine A3 receptor regulates differentiation of glioblastoma stem-like cells to endothelial cells under hypoxia. Int J Mol Sci. 2018;19(4):1228.
Ueda Y, Sakamoto N. A combined application of cyclic stretching and spheroid culture promotes endothelial differentiation of mesenchymal stem cells. J Biorheol. 2023;37(2):88–95.
Riffle S, Hegde RS. Modeling tumor cell adaptations to hypoxia in multicellular tumor spheroids. J Exp Clin Cancer Res. 2017;36(1):102.
Blacher S, et al. Cell invasion in the spheroid sprouting assay: a spatial organisation analysis adaptable to cell behaviour. PLoS ONE. 2014;9(5): e97019.
Shah S, Kang K-T. Two-cell spheroid angiogenesis assay system using both endothelial colony forming cells and mesenchymal stem cells. Biomolecules Therapeutics. 2018;26(5):474.
Balcioglu HE, van de Water B, Danen EHJ. Tumor-induced remote ECM network orientation steers angiogenesis. Sci Rep. 2016;6(1):22580.
Park S, et al. One-step achievement of tumor spheroid-induced angiogenesis in a high-throughput microfluidic platform: one-step tumor angiogenesis platform. Organoid. 2023. https://doi.org/10.51335/organoid.2023.3.e3 .
Chaddad H, et al. Combining 2D angiogenesis and 3D osteosarcoma microtissues to improve vascularization. Exp Cell Res. 2017;360(2):138–45.
He YJ, et al. Immobilized RGD concentration and proteolytic degradation synergistically enhance vascular sprouting within hydrogel scaffolds of varying modulus. J Biomater Sci Polym Ed. 2020;31(3):324–49.
Vakhrushev IV, et al. Heterotypic multicellular spheroids as experimental and preclinical models of sprouting angiogenesis. Biology (Basel). 2021;11(1):18.
Choi J, et al. FGF2-primed 3D spheroids producing IL-8 promote therapeutic angiogenesis in murine hindlimb ischemia. npj Regenerative Med. 2021;6(1):48.
Brüningk SC, et al. A cellular automaton model for spheroid response to radiation and hyperthermia treatments. Sci Rep. 2019;9(1):17674.
de Oliveira Silva N, et al. Cellular and molecular antiproliferative effects in 2D monolayer and 3D-cultivated HT-29 cells treated with zerumbone. Naunyn-Schmiedeberg’s Arch Pharmacol. 2023;397(3):1561–73.
Brüningk SC, et al. 3D tumour spheroids for the prediction of the effects of radiation and hyperthermia treatments. Sci Rep. 2020;10(1):1653.
Szade K, et al. Spheroid-plug model as a tool to study tumor development, angiogenesis, and heterogeneity in vivo. Tumor Biology. 2016;37(2):2481–96.
Durymanov M, et al. Subcutaneous inoculation of 3D pancreatic cancer spheroids results in development of reproducible stroma-rich tumors. Transl Oncol. 2019;12(1):180–9.
Tachibana T, et al. Establishment of an in vivo xenograft mouse model of a subcutaneous submillimeter HT-29 tumor formed from a single spheroid transplanted using radiation-crosslinked gelatin hydrogel microwell. Appl Sci. 2021. https://doi.org/10.3390/app11157031 .
Wang T, et al. Antioxidants stimulate BACH1-dependent tumor angiogenesis. J Clin Invest. 2023. https://doi.org/10.1172/JCI169671 .
Choi SY, et al. Differential angiogenic potential of 3-dimension spheroid of HNSCC cells in mouse xenograft. Int J Mol Sci. 2021;22(15):8245.
Izadpanah M, et al. Exosomes as theranostic agents in reproduction system. Adv Biol. 2023;8(2):2300258.
Ateeq M, et al. Extracellular vesicles&rsquo role in angiogenesis and altering angiogenic signaling. Med Sci. 2024. https://doi.org/10.3390/medsci12010004 .
Pereira RC, et al. Elucidating the role of matrix porosity and rigidity in glioblastoma type IV progression. Appl Sci. 2020. https://doi.org/10.3390/app10249076 .
Johnson PA, et al. A rapid high throughput bioprinted colorectal cancer spheroid platform for in vitro drug- and radiation-response. Biofabrication. 2023;15(1): 014103.
Fiore PF, et al. Different effects of NK cells and NK-derived soluble factors on cell lines derived from primary or metastatic pancreatic cancers. Cancer Immunol Immunother. 2023;72(6):1417–28.
Capik O, Gumus R, Karatas OF. Hypoxia-induced tumor exosomes promote angiogenesis through miR-1825/TSC2/mTOR axis in oral squamous cell carcinoma. Head Neck. 2023;45(9):2259–73.
Zhou Y, et al. Bone marrow mesenchymal stem cells-derived exosomes for penetrating and targeted chemotherapy of pancreatic cancer. Acta Pharm Sin B. 2020;10(8):1563–75.
Hao R, et al. A high-throughput nanofluidic device for exosome nanoporation to develop cargo delivery vehicles. Small. 2021;17(35):2102150.
Anthon SG, Valente KP. Vascularization strategies in 3D cell culture models: from scaffold-free models to 3D bioprinting. Int J Mol Sci. 2022;23(23):14582.
Liu C, et al. Droplet-based bioprinting for fabrication of tumor spheroids. Int J Bioprinting. 2024;10(1):1214.
Utama RH, et al. A 3D bioprinter specifically designed for the high-throughput production of matrix-embedded multicellular spheroids. Iscience. 2020. https://doi.org/10.1016/j.isci.2020.101621 .
Hong S, Song JM. 3D bioprinted drug-resistant breast cancer spheroids for quantitative in situ evaluation of drug resistance. Acta Biomater. 2022;138:228–39.
Wang X, et al. Converging bioprinting and organoids to better recapitulate the tumor microenvironment. Trends Biotechnol. 2023;42:648.
Meng F, et al. 3D bioprinted in vitro metastatic models via reconstruction of tumor microenvironments. Adv Mater. 2019;31(10):1806899.
Ko J, et al. Patient-derived tumor spheroid-induced angiogenesis preclinical platform for exploring therapeutic vulnerabilities in cancer. Biomaterials. 2024;306:122504.
Han S, et al. 3D bioprinted vascularized tumour for drug testing. Int J Mol Sci. 2020;21(8):2993.
Li X, et al. Microfluidic 3D cell culture: potential application for tissue-based bioassays. Bioanalysis. 2012;4(12):1509–25.
Miller JS, et al. Rapid casting of patterned vascular networks for perfusable engineered three-dimensional tissues. Nat Mater. 2012;11(9):768–74.
Wan Z, et al. New strategy for promoting vascularization in tumor spheroids in a microfluidic assay. Adv Healthcare Mater. 2023;12(14):2201784.
Ngo MT, Sarkaria JN, Harley BAC. Perivascular stromal cells instruct glioblastoma invasion, proliferation, and therapeutic response within an engineered brain perivascular niche model. Adv Sci. 2022;9(31):2201888.
Mitrakas AG, et al. Applications and advances of multicellular tumor spheroids: challenges in their development and analysis. Int J Molecular Sci. 2023;24(8):6949.
Langhans SA. Three-dimensional in vitro cell culture models in drug discovery and drug repositioning. Front Pharmacol. 2018;23(9):6.
Han SJ, Kwon S, Kim KS. Challenges of applying multicellular tumor spheroids in preclinical phase. Cancer Cell Int. 2021;21:1–9.
Achilli TM, Meyer J, Morgan JR. Advances in the formation, use and understanding of multi-cellular spheroids. Expert Opin Biol Therapy. 2012;12(10):1347–60.
Serra D, et al. Self-organization and symmetry breaking in intestinal organoid development. Nature. 2019;569(7754):66–72.
Kim S, et al. Uniform sized cancer spheroids production using hydrogel-based droplet microfluidics: a review. Biomed Microdevices. 2024;26(2):1–9.
Franchi-Mendes T, et al. 3D cancer models: depicting cellular crosstalk within the tumour microenvironment. Cancers. 2021;13(18):4610.
Qu J, et al. Tumor organoids: synergistic applications, current challenges, and future prospects in cancer therapy. Cancer Commun. 2021;41(12):1331–53.
Marques dos Reis E, Vieira Berti F. Vasculogenic mimicry—an overview. In: Marques dos Reis E, Berti F, editors. Vasculogenic mimicry. Methods in molecular biology, vol. 2514. New York: Humana; 2022.
Chapter Google Scholar
Bidan N, et al. Multicellular tumor spheroid model to study the multifaceted role of tumor-associated macrophages in PDAC. Drug Deliv Transl Res. 2024;14(8):2085–99.
Nashimoto Y, et al. Vascularized cancer on a chip: the effect of perfusion on growth and drug delivery of tumor spheroid. Biomaterials. 2020;229: 119547.
Nii T, Makino K, Tabata YJC. Three-dimensional culture system of cancer cells combined with biomaterials for drug screening. Cancers. 2020;12(10):2754.
Yao L, et al. Application of tumoroids derived from advanced colorectal cancer patients to predict individual response to chemotherapy. J Chemother. 2023;35(2):104–16.
Powley IR, et al. Patient-derived explants (PDEs) as a powerful preclinical platform for anti-cancer drug and biomarker discovery. Br J Cancer. 2020;122(6):735–44.
Quarta A, et al. Investigation on the composition of agarose-collagen I blended hydrogels as matrices for the growth of spheroids from breast cancer cell lines. Pharmaceutics. 2021;13(7):963.
Kim W, et al. Therapeutic strategies of three-dimensional stem cell spheroids and organoids for tissue repair and regeneration. Bioactive Mater. 2023;19:50–74.
Chai Q, Jiao Y, Yu X. Hydrogels for biomedical applications: their characteristics and the mechanisms behind them. Gels. 2017;3(1):6.
Sepantafar M, et al. Engineered hydrogels in cancer therapy and diagnosis. Trends Biotechnol. 2017;35(11):1074–87.
Spicer CD. Hydrogel scaffolds for tissue engineering: the importance of polymer choice. Polym Chem. 2020;11(2):184–219.
Emmermacher J, et al. Engineering considerations on extrusion-based bioprinting: interactions of material behavior, mechanical forces and cells in the printing needle. Biofabrication. 2020;12(2): 025022.
Leiva MC, et al. Breast cancer patient-derived scaffolds as a tool to monitor chemotherapy responses in human tumor microenvironments. J Cell Physiol. 2021;236(6):4709–24.
Parkinson GT, et al. Patient-derived scaffolds as a model of colorectal cancer. Cancer Med. 2021;10(3):867–82.
Permlid AM, et al. Unique animal friendly 3D culturing of human cancer and normal cells. Toxicol In Vitro. 2019;60:51–60.
Dondajewska E, et al. Heterotypic breast cancer model based on a silk fibroin scaffold to study the tumor microenvironment. Oncotarget. 2018;9(4):4935.
Xue J, et al. States U. Electrospinning and electrospun nano fibers: methods, materials, and applications. Chem Rev. 2019;119:5298.
Kuriakose AE, et al. Scaffold-based lung tumor culture on porous PLGA microparticle substrates. PLoS ONE. 2019;14(5): e0217640.
Bosnakovski D, et al. Chondrogenic differentiation of bovine bone marrow mesenchymal stem cells in pellet cultural system. Exp Hematol. 2004;32(5):502–9.
Zhang L, et al. Chondrogenic differentiation of human mesenchymal stem cells: a comparison between micromass and pellet culture systems. Biotech Lett. 2010;32:1339–46.
Caron MM, et al. Redifferentiation of dedifferentiated human articular chondrocytes: comparison of 2D and 3D cultures. Osteoarthritis Cartilage. 2012;20(10):1170–8.
Francioli SE, et al. Effect of three-dimensional expansion and cell seeding density on the cartilage-forming capacity of human articular chondrocytes in type II collagen sponges. J Biomed Mater Res Part A. 2010;95(3):924–31.
Loeser RF, et al. Osteoarthritis: a disease of the joint as an organ. Arthritis Rheum. 2012;64(6):1697.
Schulze-Tanzil G, et al. Redifferentiation of dedifferentiated human chondrocytes in high-density cultures. Cell Tissue Res. 2002;308:371–9.
Zhang L, et al. Chondrogenic differentiation of human mesenchymal stem cells: a comparison between micromass and pellet culture systems. Biotechnol Lett. 2010;32(9):1339–46.
Rai MF, et al. Molecular and phenotypic modulations of primary and immortalized canine chondrocytes in different culture systems. Res Vet Sci. 2009;87(3):399–407.
Fickert S, et al. One-year clinical and radiological results of a prospective, investigator-initiated trial examining a novel, purely autologous 3-dimensional autologous chondrocyte transplantation product in the knee. Cartilage. 2012;3(1):27–42.
Raffo-Romero A, et al. Establishment and characterization of canine mammary tumoroids for translational research. BMC Biol. 2023;21(1):23.
Zhu X, et al. Cancer evolution: a means by which tumors evade treatment. Biomed Pharmacother. 2021;133: 111016.
Timmins NE, Nielsen LK. Generation of multicellular tumor spheroids by the hanging-drop method. In: Hauser H, Fussenegger M, editors. Tissue engineering, vol. 140. New York: Humana Press; 2007.
Costa EC, et al. Spheroids formation on non-adhesive surfaces by liquid overlay technique: considerations and practical approaches. Biotechnol J. 2018;13(1):1700417.
Bhatia SN, Ingber DE. Microfluidic organs-on-chips. Nat Biotechnol. 2014;32(8):760–72.
Huh D. A human breathing lung-on-a-chip. Ann Am Thorac Soc. 2015;12(Supplement 1):S42–4.
Shrestha J, et al. Lung-on-a-chip: the future of respiratory disease models and pharmacological studies. Crit Rev Biotechnol. 2020;40(2):213–30.
Jang K-J, et al. Reproducing human and cross-species drug toxicities using a Liver-Chip. Sci Transl Med. 2019;11(517):eaax5516.
Dickson I. Multispecies liver-on-a-chip for improved drug toxicity testing. Nat Rev Gastroenterol Hepatol. 2020;17(1):4–4.
Wilmer MJ, et al. Kidney-on-a-chip technology for drug-induced nephrotoxicity screening. Trends Biotechnol. 2016;34(2):156–70.
Abulaiti M, et al. Establishment of a heart-on-a-chip microdevice based on human iPS cells for the evaluation of human heart tissue function. Sci Rep. 2020;10(1):19201.
Sirenko O et al. Evaluating Drug Response in 3D Triple Negative Breast Cancer Tumoroids with High Content Imaging and Analysis. 2022.
Melissaridou S, et al. The effect of 2D and 3D cell cultures on treatment response, EMT profile and stem cell features in head and neck cancer. Cancer Cell Int. 2019;19:16.
El Feky SE, et al. Cytotoxic, chemosensitizing and radiosensitizing effects of curcumin based on thioredoxin system inhibition in breast cancer cells: 2D vs. 3D cell culture system. Exp Ther Med. 2021;21(5):506.
Chen Z, et al. 3D hanging spheroid plate for high-throughput CAR T cell cytotoxicity assay. J Nanobiotechnol. 2022;20(1):30.
Zhao L, et al. A 3D printed hanging drop dripper for tumor spheroids analysis without recovery. Sci Rep. 2019;9(1):19717.
Kroupová J, Hanuš J, Štěpánek F. Surprising efficacy twist of two established cytostatics revealed by a-la-carte 3D cell spheroid preparation protocol. Eur J Pharm Biopharm. 2022;180:224–37.
Sokolova V, et al. Calcium phosphate nanoparticle-mediated transfection in 2D and 3D mono- and co-culture cell models. Acta Biomater. 2019;84:391–401.
Sarkar S, Peng CC, Tung YC. Comparison of VEGF-A secretion from tumor cells under cellular stresses in conventional monolayer culture and microfluidic three-dimensional spheroid models. PLoS ONE. 2020;15(11): e0240833.
Stryker ZI, et al. Evaluation of angiogenesis assays. Biomedicines. 2019;7(2):37.
Katt ME, et al. In vitro tumor models: advantages, disadvantages, variables, and selecting the right platform. Front Bioeng Biotechnol. 2016;4:12.
Carpentier G, et al. Angiogenesis analyzer for imageJ—a comparative morphometric analysis of “endothelial tube formation assay” and “fibrin bead assay.” Sci Rep. 2020;10(1):11568.
Xie D, et al. Effects of dulaglutide on endothelial progenitor cells and arterial elasticity in patients with type 2 diabetes mellitus. Cardiovasc Diabetol. 2022;21(1):200.
Khoo CP, Micklem K, Watt SM. A comparison of methods for quantifying angiogenesis in the Matrigel assay in vitro. Tissue Eng Part C Methods. 2011;17(9):895–906.
Nowak-Sliwinska P, et al. Consensus guidelines for the use and interpretation of angiogenesis assays. Angiogenesis. 2018;21(3):425–532.
Mason J, Öhlund D. Key aspects for conception and construction of co-culture models of tumor-stroma interactions. Front Bioeng Biotechnol. 2023;11:1150764.
Truelsen SLB, et al. The cancer angiogenesis co-culture assay: In vitro quantification of the angiogenic potential of tumoroids. PLoS ONE. 2021;16(7): e0253258.
Li S, et al. Identification of angiogenesis inhibitors using a co-culture cell model in a high-content and high-throughput screening platform. SLAS Technol. 2018;23(3):217–25.
Lin S, He X, He Y. Co-culture of ASCs/EPCs and dermal extracellular matrix hydrogel enhances the repair of full-thickness skin wound by promoting angiogenesis. Stem Cell Res Ther. 2021;12(1):129.
Perry L, et al. Co-culture systems for vasculogenesis. In: Holnthoner W, et al., editors. Vascularization for tissue engineering and regenerative medicine. Cham: Springer; 2021. p. 385–413.
Simons M, et al. State-of-the-art methods for evaluation of angiogenesis and tissue vascularization: a scientific statement from the american heart association. Circ Res. 2015;116(11):e99-132.
Chu P-Y, et al. Applications of the chick chorioallantoic membrane as an alternative model for cancer studies. Cells Tissues Organs. 2021;211(2):222–37.
Janser FA, et al. The Chick Chorioallantoic Membrane (CAM) Assay as a Three-dimensional Model to Study Autophagy in Cancer Cells. Bio Protoc. 2019;9(13): e3290.
Stoletov K, et al. Discovery of metastatic regulators using a rapid and quantitative intravital chick chorioallantoic membrane model. J Vis Exp. 2021;168: e62077.
Ribatti D. The CAM assay in the study of the metastatic process. Exp Cell Res. 2021;400(2): 112510.
Merckx G, et al. Chorioallantoic membrane assay as model for angiogenesis in tissue engineering: focus on stem cells. Tissue Eng Part B Rev. 2020;26(6):519–39.
Birsner AE, Benny O, D’Amato RJ. The corneal micropocket assay: a model of angiogenesis in the mouse eye. J Vis Exp. 2014;90:e51375.
Morbidelli L, Ciccone V, Ziche M. Studying angiogenesis in the rabbit corneal pocket assay. In: Ribatti D, editor. Vascular morphogenesis: methods and protocols. New York: Springer; 2021. p. 89–101.
Mir B. Laboratory study on the effect of plastic waste additive on shear strength of marginal soil. In: Sustainable Civil Engineering Practices: Select Proceedings of ICSCEP 2019. 2020. Springer.
Aref Z, Quax PHA. In vivo matrigel plug assay as a potent method to investigate specific individual contribution of angiogenesis to blood flow recovery in mice. Int J Mol Sci. 2021;22(16):8909.
Cabezas-Sáinz P, et al. Modeling cancer using zebrafish xenografts: drawbacks for mimicking the human microenvironment. Cells. 2020;9(9):1978.
Eberlein J, et al. Molecular and cellular mechanisms of vascular development in Zebrafish. Life (Basel). 2021;11(10):1088.
Makwana S, Mandal CC. Animal models for angiogenesis on cancer research. In: Pathak S, Banerjee A, Bisgin A, editors. Handbook of animal models and its uses in cancer research. Singapore: Springer; 2023. p. 397–419.
Kapoor A, Chen CG, Iozzo RV. A simplified aortic ring assay: a useful ex vivo method to assess biochemical and functional parameters of angiogenesis. Matrix Biol Plus. 2020;6–7: 100025.
Iqbal F, et al. Angiogenic potency evaluation of cell therapy candidates by a novel application of the in vitro aortic ring assay. Stem Cell Res Ther. 2017;8(1):184.
Kim SJ, et al. Molecular, cellular, and functional heterogeneity of retinal and choroidal endothelial cells. Invest Ophthalmol Vis Sci. 2023;64(10):35–35.
Tomita Y, et al. An ex vivo choroid sprouting assay of ocular microvascular angiogenesis. J Vis Exp. 2020;162:e61677.
Tetzlaff F, Fischer A. Human endothelial cell spheroid-based sprouting angiogenesis assay in collagen. Bio Protoc. 2018;8(17): e2995.
Augustin H, Korff T. SP0090 3D spheroids for the study of endothelial cell differentiation and angiogenesis. London: BMJ Publishing Group Ltd.; 2001.
Book Google Scholar
Vorwald CE, Joshee S, Leach JK. Spatial localization of endothelial cells in heterotypic spheroids influences Notch signaling. J Mol Med. 2020;98(3):425–35.
Bauman E, et al. Xeno-free pre-vascularized spheroids for therapeutic applications. Sci Rep. 2018;8(1):230.
Eckermann CW, et al. Characterization and modulation of fibroblast/endothelial cell co-cultures for the in vitro preformation of three-dimensional tubular networks. Cell Biol Int. 2011;35(11):1097–110.
Dey M, et al. Studying tumor angiogenesis and cancer invasion in a three-dimensional vascularized breast cancer micro-environment. Adv Biol (Weinh). 2021;5(7): e2100090.
Park S, et al. One-step achievement of tumor spheroid-induced angiogenesis in a high-throughput microfluidic platform: one-step tumor angiogenesis platform. Organoid. 2023;3: e3.
Wartenberg M, et al. Tumor-induced angiogenesis studied in confrontation cultures of multicellular tumor spheroids and embryoid bodies grown from pluripotent embryonic stem cells. FASEB J. 2001;15(6):995–1005.
Chaddad H. Development of vascularized tumor spheroids mimicking the tumor environment: angiogenesis and hypoxia. 2019, Strasbourg.
Timmins N, Dietmair S, Nielsen L. Hanging-drop multicellular spheroids as a model of tumour angiogenesis. Angiogenesis. 2004;7:97–103.
Franchi-Mendes T, Lopes N, Brito C. Heterotypic tumor spheroids in agitation-based cultures: a scaffold-free cell model that sustains long-term survival of endothelial cells. Front Bioeng Biotechnol. 2021;9:649949.
Ko J, et al. Tumor spheroid-on-a-chip: a standardized microfluidic culture platform for investigating tumor angiogenesis. Lab Chip. 2019;19(17):2822–33.
Tae Joon K and Esak L, <em>In vitro</em> modeling of tumor spheroid interactions to perfused blood vessels. bioRxiv, 2020; 2020.08.03.234633.
Filho IPT, Hartley-Asp B, Borgström P. Quantitative angiogenesis in a syngeneic tumor spheroid model. Microvasc Res. 1995;49(2):212–26.
Alajati A, et al. Spheroid-based engineering of a human vasculature in mice. Nat Methods. 2008;5(5):439–45.
Bingle L, et al. Macrophages promote angiogenesis in human breast tumour spheroids in vivo. Br J Cancer. 2006;94(1):101–7.
Download references
Acknowledgements
Authors wish to thank the personnel of the Stem Cell Research Center for their help and guidance.
This study was supported by a grant (no: 72983) from Tabriz University of Medical Sciences under the ethical code of IR.TBZMED.VCR.REC.1402.216.
Author information
Zahra Abbasi-Malati and Parisa Khanicheragh have equally contribution to this work.
Authors and Affiliations
Student Research Committee, Tabriz University of Medical Sciences, Tabriz, Iran
Zahra Abbasi-Malati
Stem Cell Research Center, Tabriz University of Medical Sciences, Tabriz, Iran
Parisa Khanicheragh, Maryam Taghavi Narmi, Fatemeh Sadeghsoltani, Sara Aghakhani Chegeni & Reza Rahbarghazi
Biotechnology Research Center, Tabriz University of Medical Sciences, Tabriz, Iran
Narges Mardi
Stem Cell and Tissue Engineering Research Laboratory, Sahand University of Technology, Tabriz, 51335-1996, Iran
Nafiseh Didar Khosrowshahi
Pediatric Health Research Center, Tabriz University of Medical Sciences, Tabriz, Iran
Amirataollah Hiradfar
Cardiovascular Research Center, Tabriz University of Medical Sciences, Tabriz, Iran
Aysa Rezabakhsh
Department of Medical Biotechnology, Faculty of Medicine, Zanjan University of Medical Sciences, Zanjan, Iran
Somayyeh Rashidi
Department of Plant, Cell and Molecular Biology, Faculty of Natural Sciences, University of Tabriz, Tabriz, Iran
Golbarg Roozbahani
Department of Applied Cell Sciences, Faculty of Advanced Medical Sciences, Tabriz University of Medical Sciences, Tabriz, Iran
Reza Rahbarghazi
You can also search for this author in PubMed Google Scholar
Contributions
Z. A-M., P.K., M.T.N., N.M., N.D.K., A.H., A.R., F.S., S.R., S.A.C., and G. R., collected data and wrote the draft. R.R. acquired financial support and supervised the study.
Corresponding author
Correspondence to Reza Rahbarghazi .
Ethics declarations
Ethics approval and consent to participate.
Not applicable.
Consent for publication
Competing interests.
The authors declare that they have no conflict of interest.
Additional information
Publisher's note.
Springer Nature remains neutral with regard to jurisdictional claims in published maps and institutional affiliations.
Rights and permissions
Open Access This article is licensed under a Creative Commons Attribution-NonCommercial-NoDerivatives 4.0 International License, which permits any non-commercial use, sharing, distribution and reproduction in any medium or format, as long as you give appropriate credit to the original author(s) and the source, provide a link to the Creative Commons licence, and indicate if you modified the licensed material. You do not have permission under this licence to share adapted material derived from this article or parts of it. The images or other third party material in this article are included in the article’s Creative Commons licence, unless indicated otherwise in a credit line to the material. If material is not included in the article’s Creative Commons licence and your intended use is not permitted by statutory regulation or exceeds the permitted use, you will need to obtain permission directly from the copyright holder. To view a copy of this licence, visit http://creativecommons.org/licenses/by-nc-nd/4.0/ .
Reprints and permissions
About this article
Cite this article.
Abbasi-Malati, Z., Khanicheragh, P., Narmi, M.T. et al. Tumoroids, a valid preclinical screening platform for monitoring cancer angiogenesis. Stem Cell Res Ther 15 , 267 (2024). https://doi.org/10.1186/s13287-024-03880-4
Download citation
Received : 25 February 2024
Accepted : 06 August 2024
Published : 26 August 2024
DOI : https://doi.org/10.1186/s13287-024-03880-4
Share this article
Anyone you share the following link with will be able to read this content:
Sorry, a shareable link is not currently available for this article.
Provided by the Springer Nature SharedIt content-sharing initiative
- Cancer angiogenesis assay
Stem Cell Research & Therapy
ISSN: 1757-6512
- Submission enquiries: Access here and click Contact Us
- General enquiries: [email protected]
Information
- Author Services
Initiatives
You are accessing a machine-readable page. In order to be human-readable, please install an RSS reader.
All articles published by MDPI are made immediately available worldwide under an open access license. No special permission is required to reuse all or part of the article published by MDPI, including figures and tables. For articles published under an open access Creative Common CC BY license, any part of the article may be reused without permission provided that the original article is clearly cited. For more information, please refer to https://www.mdpi.com/openaccess .
Feature papers represent the most advanced research with significant potential for high impact in the field. A Feature Paper should be a substantial original Article that involves several techniques or approaches, provides an outlook for future research directions and describes possible research applications.
Feature papers are submitted upon individual invitation or recommendation by the scientific editors and must receive positive feedback from the reviewers.
Editor’s Choice articles are based on recommendations by the scientific editors of MDPI journals from around the world. Editors select a small number of articles recently published in the journal that they believe will be particularly interesting to readers, or important in the respective research area. The aim is to provide a snapshot of some of the most exciting work published in the various research areas of the journal.
Original Submission Date Received: .
- Active Journals
- Find a Journal
- Proceedings Series
- For Authors
- For Reviewers
- For Editors
- For Librarians
- For Publishers
- For Societies
- For Conference Organizers
- Open Access Policy
- Institutional Open Access Program
- Special Issues Guidelines
- Editorial Process
- Research and Publication Ethics
- Article Processing Charges
- Testimonials
- Preprints.org
- SciProfiles
- Encyclopedia
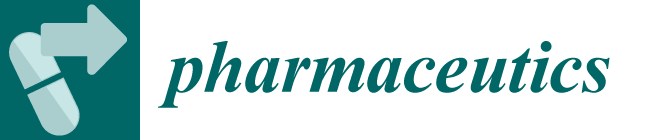
Article Menu
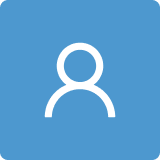
- Subscribe SciFeed
- Recommended Articles
- Google Scholar
- on Google Scholar
- Table of Contents
Find support for a specific problem in the support section of our website.
Please let us know what you think of our products and services.
Visit our dedicated information section to learn more about MDPI.
JSmol Viewer
Her-2 receptor and αvβ3 integrin dual-ligand surface-functionalized liposome for metastatic breast cancer therapy.
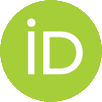
Graphical Abstract
1. Introduction
2. materials and methods, 2.1. materials, 2.2. methods, 2.2.1. synthesis of tpgs-cooh, 2.2.2. synthesis of trast-tpgs-cooh hybrid conjugate, 2.2.3. irgd-tpgs-cooh hybrid conjugate, 2.2.4. experimental design and optimization of gfb and lch dual drug-loaded liposome, 2.2.5. surface functionalization of optimized liposome by irgd and trast, 3. characterization of dsdls, 3.1. zeta analysis, 3.2. scanning electron microscopy (sem) analysis, 3.3. transmission electron microscopy (tem) study, 3.4. x-ray diffraction (xrd) study, 3.5. fourier transform infrared spectrophotometer (ftir) analysis, 3.6. determination of encapsulation efficiency (ee%) and drug loading (dl%), 3.7. stability studies, 3.8. in-vitro drug release study, 3.9. blood compatibility study, 3.10. in-vitro cell study, 3.10.1. in-vitro cytotoxicity (mtt assay), 3.10.2. cellular uptake by confocal laser scanning microscopy (clsm), 3.10.3. wound-healing assay, 3.11. statistical analysis, 4.1. experimental design and optimization of liposomes, 4.1.1. bbd 3 factor-3 level approach, effect of formulation parameters (e-80, cholesterol, and tpgs) on ps, effect of formulation parameters (e-80, cholesterol, and tpgs) on pdi, effect of formulation parameters (e-80, cholesterol, and tpgs) on zp, 4.1.2. optimization of formulation by numerical analysis and point prediction, 4.2. zeta analysis, 4.3. surface morphology by sem and tem analysis, 4.4. characterization of hybrid conjugates ftir analysis, 4.4.1. characterization of tpgs-cooh by ftir, 4.4.2. characterization of irgd-tpgs-cooh by ftir, 4.4.3. characterization of trast-tpgs-cooh by ftir, 4.4.4. characterization of dsdl formulation, 4.5. ee (%) and dl (%), 4.6. in-vitro drug release profile, 4.7. xrd analysis, 4.8. stability studies, 4.9. biocompatibility study, 4.10. in-vitro cell line, 4.10.1. cytotoxicity studies, 4.10.2. cellular uptake of sdls and dsdls on sk-br-3 and mda-mb-231, 4.10.3. wound-healing assay, 5. discussion, 6. conclusions, author contributions, institutional review board statement, informed consent statement, data availability statement, acknowledgments, conflicts of interest, abbreviations.
HER-2 | Human epidermal growth factor receptor-2 |
Trast | Trastuzumab |
GFB | Gefitinib |
LCH | Lycorine hydrochloride |
TPGS-COOH | Activated D-Alpha-Tocopheryl polyethylene glycol 1000 succinate |
DSDL | Dual surface-decorated liposomes |
PS | Particle size |
PDI | Polydispersity index |
ZP | Zeta potential |
IC-50 | Half-maximum inhibitory concentration |
SDL | Single decorated liposomes |
CLSM | Confocal laser scanning microscopy |
ErbB-2 | Erythroblastic leukemia viral oncogene homolog-2 |
RGDK | Cryptic CendR motif |
NRP-1 | Neuropilin-1 |
TCRP1 | Tongue cancer resistance-associated protein |
Akt | Protein kinase B |
mTOR | Mammalian target of rapamycin |
BCS | Biopharmaceutical classification system |
MAPK/PI3K | Mitogen-activated protein kinase/phosphatidylinositol 3-kinase |
TME | Tumor microenvironment |
TPGS | D-Alpha-Tocopheryl polyethylene glycol 1000 succinate |
HLB | Hydrophilic and lipophilic balance |
FDA | Food and drug administration |
DSPE-PEG2000 | 1,2-distearoyl-sn-glycero-3-phosphoethanolamine-N-[amino(polyethylene glycol)-2000] |
CAFs | Cancer-associated fibroblasts |
ECM | Extracellular matrix |
TAMs | Tumor-associated macrophages |
MDSCs | Myeloid-derived suppressor cells |
TGF-β | Transforming growth factor beta |
VEGF | Vascular endothelial growth factor |
TILs | Tumor-infiltrating lymphocytes |
HIFs | Hypoxia-inducible factors |
PD-L1 | Programmed-death ligand |
CTLA-4 | Cytotoxic T-lymphocyte-associated protein-4 |
NKG-2A | Natural killer group 2A |
EDC | 1-Ethyl-3-[3-dimethylaminopropyl] carbodiimide hydrochloride |
NHS | N-hydroxy succinimide |
DMAP | 4-(Dimethyl amino) pyridine |
MTT | 3-(4,5-dimethylthiazol-2-yl)-2,5-diphenyltetrazolium bromide |
DAPI | 4′,6-diamidino-2-phenylindole dihydrochloride |
DMEM | Dulbecco’s Modified Eagle Medium |
FBS | Fetal bovine serum |
DCM | Dichloromethane |
PBS | Phosphate buffered saline |
RSM | Response surface methodology |
DOE | Design of experiments |
BBD | Box–Behnken design |
R | Correlation coefficient |
RMSE | Root mean square error |
SEM | Scanning electron microscopy |
TEM | Transmission electron microscopy |
XRD | X-ray diffraction |
FTIR | Fourier transform infrared spectroscopy |
EE | Entrapment efficiency |
DL | Drug loading |
RP-HPLC | Reverse-phase high-performance liquid chromatography |
ICH | International Council for Harmonization of Technical Requirements for Pharmaceuticals for Human Use |
ACN | Acetonitrile |
TFA | Trifluoroacetic acid |
NCCS | National Centre for Cell Science |
DMSO | Dimethyl sulfoxide |
CLSM | Confocal laser scanning microscopy |
MFI | Mean fluorescence intensity |
- Chen, G.; Wang, Y.; Wu, P.; Zhou, Y.; Yu, F.; Zhu, C.; Li, Z.; Hang, Y.; Wang, K.; Li, J.; et al. Reversibly stabilized polycation nanoparticles for combination treatment of early-and late-stage metastatic breast cancer. ACS Nano 2018 , 12 , 6620–6636. [ Google Scholar ] [ CrossRef ] [ PubMed ]
- Kim, J.; Lee, C.; Kim, I.; Ro, J.; Kim, J.; Min, Y.; Park, J.; Sunkara, V.; Park, Y.-S.; Michael, I.; et al. Three-dimensional human liver-chip emulating premetastatic niche formation by breast cancer-derived extracellular vesicles. ACS Nano 2020 , 14 , 14971–14988. [ Google Scholar ] [ CrossRef ] [ PubMed ]
- Cao, H.; Dan, Z.; He, X.; Zhang, Z.; Yu, H.; Yin, Q.; Li, Y. Liposomes coated with isolated macrophage membrane can target lung metastasis of breast cancer. ACS Nano 2016 , 10 , 7738–7748. [ Google Scholar ] [ CrossRef ]
- Pandey, P.; Arya, D.K.; Ramar, M.K.; Chidambaram, K.; Rajinikanth, P. Engineered nanomaterials as an effective tool for HER2+ breast cancer therapy. Drug Discov. Today 2022 , 27 , 2526–2540. [ Google Scholar ] [ CrossRef ] [ PubMed ]
- Zi, Y.; Yang, K.; He, J.; Wu, Z.; Liu, J.; Zhang, W. Strategies to enhance drug delivery to solid tumors by harnessing the EPR effects and alternative targeting mechanisms. Adv. Drug Deliv. Rev. 2022 , 188 , 114449. [ Google Scholar ] [ CrossRef ]
- Huang, D.; Sun, L.; Huang, L.; Chen, Y. Nanodrug delivery systems modulate tumor vessels to increase the enhanced permeability and retention effect. J. Pers. Med. 2021 , 11 , 124. [ Google Scholar ] [ CrossRef ]
- Piccart-Gebhart, M.J.; Procter, M.; Leyland-Jones, B.; Goldhirsch, A.; Untch, M.; Smith, I.; Gianni, L.; Baselga, J.; Bell, R.; Jackisch, C.; et al. Trastuzumab after adjuvant chemotherapy in HER2-positive breast cancer. N. Engl. J. Med. 2005 , 353 , 1659–1672. [ Google Scholar ] [ CrossRef ]
- Loibl, S.; Gianni, L. HER2-positive breast cancer. Lancet 2017 , 389 , 2415–2429. [ Google Scholar ] [ CrossRef ]
- Goldhirsch, A.; Gelber, R.D.; Piccart-Gebhart, M.J.; De Azambuja, E.; Procter, M.; Suter, T.M.; Jackisch, C.; Cameron, D.; Weber, H.A.; Heinzmann, D.; et al. 2 years versus 1 year of adjuvant trastuzumab for HER2-positive breast cancer (HERA): An open-label, randomised controlled trial. Lancet 2013 , 382 , 1021–1028. [ Google Scholar ] [ CrossRef ]
- Wang, Y.; Chen, J.; Irudayaraj, J. Nuclear targeting dynamics of gold nanoclusters for enhanced therapy of HER2+ breast cancer. ACS Nano 2011 , 5 , 9718–9725. [ Google Scholar ] [ CrossRef ]
- Slamon, D.; Eiermann, W.; Robert, N.; Pienkowski, T.; Martin, M.; Press, M.; Mackey, J.; Glaspy, J.; Chan, A.; Pawlicki, M.; et al. Adjuvant trastuzumab in HER2-positive breast cancer. N. Engl. J. Med. 2011 , 365 , 1273–1283. [ Google Scholar ] [ CrossRef ] [ PubMed ]
- Deng, Z.; Xiao, Y.; Pan, M.; Li, F.; Duan, W.; Meng, L.; Liu, X.; Yan, F.; Zheng, H. Hyperthermia-triggered drug delivery from iRGD-modified temperature-sensitive liposomes enhances the anti-tumor efficacy using high intensity focused ultrasound. J. Control. Release 2016 , 243 , 333–341. [ Google Scholar ] [ CrossRef ] [ PubMed ]
- Sonju, J.J.; Dahal, A.; Singh, S.S.; Jois, S.D. Peptide-functionalized liposomes as therapeutic and diagnostic tools for cancer treatment. J. Control. Release 2021 , 329 , 624–644. [ Google Scholar ] [ CrossRef ]
- Deepak, P.; Kumar, P.; Pandey, P.; Arya, D.K.; Jaiswal, S.; Kumar, A.; Sonkar, A.B.; Ali, D.; Alarifi, S.; Ramar, M.; et al. Pentapeptide cRGDfK-Surface Engineered Nanostructured Lipid Carriers as an Efficient Tool for Targeted Delivery of Tyrosine Kinase Inhibitor for Battling Hepatocellular Carcinoma. Int. J. Nanomed. 2023 , 18 , 7021–7046. [ Google Scholar ] [ CrossRef ] [ PubMed ]
- Deng, C.; Zhang, Q.; Fu, Y.; Sun, X.; Gong, T.; Zhang, Z. Coadministration of oligomeric hyaluronic acid-modified liposomes with tumor-penetrating peptide-iRGD enhances the antitumor efficacy of doxorubicin against melanoma. ACS Appl. Mater. Interfaces 2017 , 9 , 1280–1292. [ Google Scholar ] [ CrossRef ]
- Guan, J.; Guo, H.; Tang, T.; Wang, Y.; Wei, Y.; Seth, P.; Li, Y.; Dehm, S.M.; Ruoslahti, E.; Pang, H. iRGD-liposomes enhance tumor delivery and therapeutic efficacy of antisense oligonucleotide drugs against primary prostate cancer and bone metastasis. Adv. Funct. Mater. 2021 , 31 , 2100478. [ Google Scholar ] [ CrossRef ]
- Sun, Y.; Gu, Y.; Gao, X.; Jin, X.; Wink, M.; Sharopov, F.S.; Yang, L.; Sethi, G. Lycorine suppresses the malignancy of breast carcinoma by modulating epithelial mesenchymal transition and β-catenin signaling. Pharmacol. Res. 2023 , 195 , 106866. [ Google Scholar ] [ CrossRef ]
- Zhang, P.; Yuan, X.; Yu, T.; Huang, H.; Yang, C.; Zhang, L.; Yang, S.; Luo, X.; Luo, J. Lycorine inhibits cell proliferation, migration and invasion, and primarily exerts in vitro cytostatic effects in human colorectal cancer via activating the ROS/p38 and AKT signaling pathways. Oncol. Rep. 2021 , 45 , 19. [ Google Scholar ] [ CrossRef ]
- Zhang, W.; Yang, J.; Chen, Y.; Xue, R.; Mao, Z.; Lu, W.; Jiang, Y. Lycorine hydrochloride suppresses stress-induced premature cellular senescence by stabilizing the genome of human cells. Aging Cell 2021 , 20 , e13307. [ Google Scholar ] [ CrossRef ]
- Shi, S.; Li, C.; Zhang, Y.; Deng, C.; Tan, M.; Pan, G.; Du, J.; Ji, Y.; Li, Q.; Liang, H.; et al. Lycorine hydrochloride inhibits melanoma cell proliferation, migration and invasion via down-regulating p21Cip1/WAF1. Am. J. Cancer Res. 2021 , 11 , 1391. [ Google Scholar ]
- Li, M.-H.; Liao, X.; Li, C.; Wang, T.-T.; Sun, Y.-S.; Yang, K.; Jiang, P.-W.; Shi, S.-T.; Zhang, W.-X.; Zhang, K.; et al. Lycorine hydrochloride induces reactive oxygen species-mediated apoptosis via the mitochondrial apoptotic pathway and the JNK signaling pathway in the oral squamous cell carcinoma HSC-3 cell line. Oncol. Lett. 2021 , 21 , 236. [ Google Scholar ] [ CrossRef ] [ PubMed ]
- Li, C.; Deng, C.; Pan, G.; Wang, X.; Zhang, K.; Dong, Z.; Zhao, G.; Tan, M.; Hu, X.; Shi, S.; et al. Lycorine hydrochloride inhibits cell proliferation and induces apoptosis through promoting FBXW7-MCL1 axis in gastric cancer. J. Exp. Clin. Cancer Res. 2020 , 39 , 230. [ Google Scholar ] [ CrossRef ] [ PubMed ]
- Wang, J.; Xu, J.; Xing, G. Lycorine inhibits the growth and metastasis of breast cancer through the blockage of STAT3 signaling pathway. Acta Biochim. Biophys. Sin. 2017 , 49 , 771–779. [ Google Scholar ] [ CrossRef ] [ PubMed ]
- Nayek, S.; Raghavendra, N.; Kumar, B.S. Development of novel S PC-3 gefitinib lipid nanoparticles for effective drug delivery in breast cancer. Tissue distribution studies and cell cytotoxicity analysis. J. Drug Deliv. Sci. Technol. 2021 , 61 , 102073. [ Google Scholar ] [ CrossRef ]
- Lin, K.-H.; Hong, S.-T.; Wang, H.-T.; Lo, Y.-L.; Lin, A.M.-Y.; Yang, J.C.-H. Enhancing anticancer effect of gefitinib across the blood–brain barrier model using liposomes modified with one α-helical cell-penetrating peptide or glutathione and tween 80. Int. J. Mol. Sci. 2016 , 17 , 1998. [ Google Scholar ] [ CrossRef ]
- Takenaka, T.; Nakai, S.; Katayama, M.; Hirano, M.; Ueno, N.; Noguchi, K.; Takatani-Nakase, T.; Fujii, I.; Kobayashi, S.S.; Nakase, I. Effects of gefitinib treatment on cellular uptake of extracellular vesicles in EGFR-mutant non-small cell lung cancer cells. Int. J. Pharm. 2019 , 572 , 118762. [ Google Scholar ] [ CrossRef ]
- Sitia, L.; Sevieri, M.; Signati, L.; Bonizzi, A.; Chesi, A.; Mainini, F.; Corsi, F.; Mazzucchelli, S. HER-2-targeted nanoparticles for breast cancer diagnosis and treatment. Cancers 2022 , 14 , 2424. [ Google Scholar ] [ CrossRef ]
- Hu, C.; Liang, K.; An, R.; Wang, X.; You, L. The characterization, pharmacokinetic, and tissue distribution studies of TPGS-modified artesunate liposome in rats. Drug Dev. Ind. Pharm. 2018 , 44 , 1528–1535. [ Google Scholar ] [ CrossRef ]
- Raju, A.; Muthu, M.S.; Feng, S.-S. Trastuzumab-conjugated vitamin E TPGS liposomes for sustained and targeted delivery of docetaxel. Expert Opin. Drug Deliv. 2013 , 10 , 747–760. [ Google Scholar ] [ CrossRef ]
- Muthu, M.S.; Kulkarni, S.A.; Raju, A.; Feng, S.-S. Theranostic liposomes of TPGS coating for targeted co-delivery of docetaxel and quantum dots. Biomaterials 2012 , 33 , 3494–3501. [ Google Scholar ] [ CrossRef ]
- Assanhou, A.G.; Li, W.; Zhang, L.; Xue, L.; Kong, L.; Sun, H.; Mo, R.; Zhang, C. Reversal of multidrug resistance by co-delivery of paclitaxel and lonidamine using a TPGS and hyaluronic acid dual-functionalized liposome for cancer treatment. Biomaterials 2015 , 73 , 284–295. [ Google Scholar ] [ CrossRef ]
- Vijayakumar, M.R.; Vajanthri, K.Y.; Balavigneswaran, C.K.; Mahto, S.K.; Mishra, N.; Muthu, M.S.; Singh, S. Pharmacokinetics, biodistribution, in vitro cytotoxicity and biocompatibility of Vitamin E TPGS coated trans resveratrol liposomes. Colloids Surf. B Biointerfaces 2016 , 145 , 479–491. [ Google Scholar ] [ CrossRef ]
- Farooq, M.A.; Xinyu, H.; Jabeen, A.; Ahsan, A.; Seidu, T.A.; Kutoka, P.T.; Wang, B. Enhanced cellular uptake and cytotoxicity of vorinostat through encapsulation in TPGS-modified liposomes. Colloids Surf. B Biointerfaces 2021 , 199 , 111523. [ Google Scholar ] [ CrossRef ]
- Muthu, M.S.; Kulkarni, S.A.; Xiong, J.; Feng, S.-S. Vitamin E TPGS coated liposomes enhanced cellular uptake and cytotoxicity of docetaxel in brain cancer cells. Int. J. Pharm. 2011 , 421 , 332–340. [ Google Scholar ] [ CrossRef ]
- Pan, J.; Feng, S.-S. Targeted delivery of paclitaxel using folate-decorated poly (lactide)–vitamin E TPGS nanoparticles. Biomaterials 2008 , 29 , 2663–2672. [ Google Scholar ] [ CrossRef ]
- Kaddah, S.; Khreich, N.; Kaddah, F.; Charcosset, C.; Greige-Gerges, H. Cholesterol modulates the liposome membrane fluidity and permeability for a hydrophilic molecule. Food Chem. Toxicol. 2018 , 113 , 40–48. [ Google Scholar ] [ CrossRef ]
- Guimarães, D.; Cavaco-Paulo, A.; Nogueira, E. Design of liposomes as drug delivery system for therapeutic applications. Int. J. Pharm. 2021 , 601 , 120571. [ Google Scholar ] [ CrossRef ]
- Choi, S.; Kang, B.; Yang, E.; Kim, K.; Kwak, M.K.; Chang, P.-S.; Jung, H.-S. Precise control of liposome size using characteristic time depends on solvent type and membrane properties. Sci. Rep. 2023 , 13 , 4728. [ Google Scholar ] [ CrossRef ]
- Song, T.; Wang, H.; Liu, Y.; Cai, R.; Yang, D.; Xiong, Y. TPGS-modified long-circulating liposomes loading ziyuglycoside I for enhanced therapy of myelosuppression. Int. J. Nanomed. 2021 , 16 , 6281–6295. [ Google Scholar ] [ CrossRef ]
- Saraf, S.; Jain, A.; Tiwari, A.; Verma, A.; Panda, P.K.; Jain, S.K. Advances in liposomal drug delivery to cancer: An overview. J. Drug Deliv. Sci. Technol. 2020 , 56 , 101549. [ Google Scholar ] [ CrossRef ]
- Almeida, B.; Nag, O.K.; Rogers, K.E.; Delehanty, J.B. Recent progress in bioconjugation strategies for liposome-mediated drug delivery. Molecules 2020 , 25 , 5672. [ Google Scholar ] [ CrossRef ] [ PubMed ]
- Nel, J.; Elkhoury, K.; Velot, É.; Bianchi, A.; Acherar, S.; Francius, G.; Tamayol, A.; Grandemange, S.; Arab-Tehrany, E. Functionalized liposomes for targeted breast cancer drug delivery. Bioact. Mater. 2023 , 24 , 401–437. [ Google Scholar ] [ CrossRef ] [ PubMed ]
- Yang, D.; Liu, J.; Qian, H.; Zhuang, Q. Cancer-associated fibroblasts: From basic science to anticancer therapy. Exp. Mol. Med. 2023 , 55 , 1322–1332. [ Google Scholar ] [ CrossRef ] [ PubMed ]
- Lin, Y.; Xu, J.; Lan, H. Tumor-associated macrophages in tumor metastasis: Biological roles and clinical therapeutic applications. J. Hematol. Oncol. 2019 , 12 , 76. [ Google Scholar ] [ CrossRef ] [ PubMed ]
- Agostinetto, E.; Curigliano, G.; Piccart, M. Emerging treatments in HER2-positive advanced breast cancer: Keep raising the bar. Cell Rep. Med. 2024 , 5 , 101575. [ Google Scholar ] [ CrossRef ]
- Singh, R.P.; Sharma, G.; Kumari, L.; Koch, B.; Singh, S.; Bharti, S.; Rajinikanth, P.S.; Pandey, B.L.; Muthu, M.S. RGD-TPGS decorated theranostic liposomes for brain targeted delivery. Colloids Surf. B Biointerfaces 2016 , 147 , 129–141. [ Google Scholar ]
- Ong, T.H.; Chitra, E.; Ramamurthy, S.; Siddalingam, R.P.; Yuen, K.H.; Ambu, S.P.; Davamani, F. Chitosan-propolis nanoparticle formulation demonstrates anti-bacterial activity against Enterococcus faecalis biofilms. PLoS ONE 2017 , 12 , e0174888. [ Google Scholar ]
- Kumar, R.; Kumar, A.; Kumar, D.; Yadav, S.; Shrivastava, N.K.; Singh, J.; Sonkar, A.B.; Verma, P.; Arya, D.K.; Kaithwas, G.; et al. Harnessing Potential of ω-3 Polyunsaturated Fatty Acid with Nanotechnology for Enhanced Breast Cancer Therapy: A Comprehensive Investigation into ALA-Based Liposomal PTX Delivery. Pharmaceutics 2024 , 16 , 913. [ Google Scholar ] [ CrossRef ] [ PubMed ]
- Torres-Flores, G.; Gonzalez-Horta, A.; Vega-Cantu, Y.I.; Rodriguez, C.; Rodriguez-Garcia, A. Preparation and Characterization of Liposomal Everolimus by Thin-Film Hydration Technique. Adv. Polym. Technol. 2020 , 2020 , 5462949. [ Google Scholar ] [ CrossRef ]
- Jaiswal, S.; Anjum, M.M.; Arya, D.K.; Thakur, S.; Pandey, P.; Deepak, P.; Kanaujiya, S.; Anand, S.; Kaushik, A.S.; Mishra, V.; et al. Surface entrenched β-sitosterol niosomes for enhanced cardioprotective activity against isoproterenol induced cardiotoxicity in rats. Int. J. Pharm. 2024 , 653 , 123872. [ Google Scholar ] [ CrossRef ] [ PubMed ]
- Rajinikanth, P.; Sankar, C.; Mishra, B. Sodium alginate microspheres of metoprolol tartrate for intranasal systemic delivery: Development and evaluation. Drug Deliv. 2003 , 10 , 21–28. [ Google Scholar ] [ CrossRef ]
- Balasubramaniam, J.; Rao, V.U.; Vasudha, M.; Babu, J.; Rajinikanth, P. Sodium alginate microspheres of metformin HCl: Formulation and in vitro evaluation. Curr. Drug Deliv. 2007 , 4 , 249–256. [ Google Scholar ] [ CrossRef ] [ PubMed ]
- Ruozi, B.; Belletti, D.; Tombesi, A.; Tosi, G.; Bondioli, L.; Forni, F.; Vandelli, M.A. AFM, ESEM, TEM, and CLSM in liposomal characterization: A comparative study. Int. J. Nanomed. 2011 , 6 , 557–563. [ Google Scholar ] [ CrossRef ]
- Anjum, S.; Wang, Y.; Xin, Y.; Li, X.; Li, T.; Zhang, H.; Quan, L.; Li, Y.; Arya, D.K.; Rajinikanth, P.; et al. Bioinspired core-shell nanofiber drug-delivery system modulates osteogenic and osteoclast activity for bone tissue regeneration. Mater. Today Bio 2024 , 26 , 101088. [ Google Scholar ] [ CrossRef ]
- Lim, W.M.; Rajinikanth, P.S.; Mallikarjun, C.; Kang, Y.B. Formulation and delivery of itraconazole to the brain using a nanolipid carrier system. Int. J. Nanomed. 2014 , 9 , 2117–2126. [ Google Scholar ] [ CrossRef ] [ PubMed ]
- Anjum, S.; Li, T.; Arya, D.K.; Ali, D.; Alarifi, S.; Yulin, W.; Hengtong, Z.; Rajinikanth, P.; Ao, Q. Biomimetic electrospun nanofibrous scaffold for tissue engineering: Preparation, optimization by design of experiments (DOE), in-vitro and in-vivo characterization. Front. Bioeng. Biotechnol. 2023 , 11 , 1288539. [ Google Scholar ] [ CrossRef ] [ PubMed ]
- Anjum, S.; Arya, D.K.; Saeed, M.; Ali, D.; Athar, M.S.; Yulin, W.; Alarifi, S.; Wu, X.; Rajinikanth, P.; Ao, Q. Multifunctional electrospun nanofibrous scaffold enriched with alendronate and hydroxyapatite for balancing osteogenic and osteoclast activity to promote bone regeneration. Front. Bioeng. Biotechnol. 2023 , 11 , 1302594. [ Google Scholar ] [ CrossRef ] [ PubMed ]
- Bian, J.; Girotti, J.; Fan, Y.; Levy, E.S.; Zang, N.; Sethuraman, V.; Kou, P.; Zhang, K.; Gruenhagen, J.; Lin, J. Fast and versatile analysis of liposome encapsulation efficiency by nanoParticle exclusion chromatography. J. Chromatogr. A 2022 , 1662 , 462688. [ Google Scholar ] [ CrossRef ]
- Ohnishi, N.; Yamamoto, E.; Tomida, H.; Hyodo, K.; Ishihara, H.; Kikuchi, H.; Tahara, K.; Takeuchi, H. Rapid determination of the encapsulation efficiency of a liposome formulation using column-switching HPLC. Int. J. Pharm. 2013 , 441 , 67–74. [ Google Scholar ] [ CrossRef ]
- Rajinikanth, P.; Keat, N.W.; Garg, S. Self-nanoemulsifying drug delivery systems of Valsartan: Preparation and in-vitro characterization. Int. J. Drug Deliv. 2012 , 4 , 153. [ Google Scholar ]
- Rajinikanth, P.S.; Karunagaran, L.N.; Balasubramaniam, J.; Mishra, B. Formulation and evaluation of clarithromycin microspheres for eradication of Helicobacter pylori. Chem. Pharm. Bull. 2008 , 56 , 1658–1664. [ Google Scholar ] [ CrossRef ] [ PubMed ]
- Muppidi, K.; Pumerantz, A.S.; Wang, J.; Betageri, G. Development and Stability Studies of Novel Liposomal Vancomycin Formulations. ISRN Pharm. 2012 , 2012 , 636743. [ Google Scholar ] [ CrossRef ]
- Taira, M.C.; Chiaramoni, N.S.; Pecuch, K.M.; Alonso-Romanowski, S. Stability of Liposomal Formulations in Physiological Conditions for Oral Drug Delivery. Drug Deliv. 2004 , 11 , 123–128. [ Google Scholar ] [ CrossRef ]
- Rajinikanth, P.S.; Mishra, B. Stomach-site specific drug delivery system of clarithromycin for eradication of Helicobacter pylori. Chem. Pharm. Bull. 2009 , 57 , 1068–1075. [ Google Scholar ] [ CrossRef ] [ PubMed ]
- Panwar, P.; Pandey, B.; Lakhera, P.C.; Singh, K.P. Preparation, characterization, and in vitro release study of albendazole-encapsulated nanosize liposomes. Int. J. Nanomed. 2010 , 5 , 101–108. [ Google Scholar ] [ CrossRef ]
- Rajinikanth, P.S.; Chellian, J. Development and evaluation of nanostructured lipid carrier-based hydrogel for topical delivery of 5-fluorouracil. Int. J. Nanomed. 2016 , 11 , 5067–5077. [ Google Scholar ] [ CrossRef ]
- Jaiswal, S.; Anjum, M.M.; Thakur, S.; Pandey, P.; Arya, D.K.; Kumar, A.; Kaushik, A.S.; Rajinikanth, P.S. Evaluation of cardioprotective effect of naringin loaded lignin nanoparticles against isoproterenol induced myocardial infarction. J. Drug Deliv. Sci. Technol. 2023 , 89 , 105076. [ Google Scholar ] [ CrossRef ]
- Maurelli, A.M.; De Leo, V.; Daniello, V.; Calvano, C.D.; Ciriaco, F.; Milano, F.; Ingrosso, C.; Cataldi, T.R.; Di Gioia, S.; Conese, M.; et al. In depth study of the polydopamine coating of liposomes as a potential alternative to PEGylation for the stabilization of nanocarriers in biological fluids. Mater. Today Chem. 2024 , 37 , 101994. [ Google Scholar ] [ CrossRef ]
- Wang, P.; Zhong, W.; Huang, Q.; Zhu, Y.; Chen, L.; Ye, K. Liposome Nanomedicine Based on Tumor Cell Lysate Mitigates the Progression of Lynch Syndrome-Associated Colon Cancer. ACS Biomater. Sci. Eng. 2024 , 10 , 3136–3147. [ Google Scholar ] [ CrossRef ]
- Kanaujiya, S.; Arya, D.K.; Pandey, P.; Singh, S.; Pandey, G.; Anjum, S.; Anjum, M.M.; Ali, D.; Alarifi, S.; Vijayakumar, M.R.; et al. Resveratrol-Ampicillin Dual-Drug Loaded Polyvinylpyrrolidone/Polyvinyl Alcohol Biomimic Electrospun Nanofiber Enriched with Collagen for Efficient Burn Wound Repair. Int. J. Nanomed. 2024 , 19 , 5397–5418. [ Google Scholar ] [ CrossRef ]
- Angius, F.; Floris, A. Liposomes and MTT cell viability assay: An incompatible affair. Toxicol. In Vitro 2015 , 29 , 314–319. [ Google Scholar ] [ CrossRef ] [ PubMed ]
- Deepak, P.; Kumar, P.; Arya, D.K.; Pandey, P.; Kumar, S.; Parida, B.P.; Narayan, G.; Singh, S.; Rajinikanth, P.S. c(RGDfK) anchored surface manipulated liposome for tumor-targeted Tyrosine Kinase Inhibitor (TKI) delivery to potentiate liver anticancer activity. Int. J. Pharm. 2023 , 642 , 123160. [ Google Scholar ] [ CrossRef ] [ PubMed ]
- Bulut, G.; Atmaca, H.; Karaca, B. Trastuzumab in combination with AT-101 induces cytotoxicity and apoptosis in Her2 positive breast cancer cells. Future Oncol. 2020 , 16 , 4485–4495. [ Google Scholar ] [ CrossRef ]
- Hadisi, Z.; Farokhi, M.; Bakhsheshi-Rad, H.R.; Jahanshahi, M.; Hasanpour, S.; Pagan, E.; Dolatshahi-Pirouz, A.; Zhang, Y.S.; Kundu, S.C.; Akbari, M. Hyaluronic acid (HA)-based silk fibroin/zinc oxide core–shell electrospun dressing for burn wound management. Macromol. Biosci. 2020 , 20 , 1900328. [ Google Scholar ] [ CrossRef ]
- Mouritzen, M.V.; Jenssen, H. Optimized Scratch Assay for In Vitro Testing of Cell Migration with an Automated Optical Camera. J. Vis. Exp. JoVE 2018 , 138 , 57691. [ Google Scholar ] [ CrossRef ]
- Liang, C.C.; Park, A.Y.; Guan, J.L. In vitro scratch assay: A convenient and inexpensive method for analysis of cell migration in vitro. Nat. Protoc. 2007 , 2 , 329–333. [ Google Scholar ] [ CrossRef ] [ PubMed ]
- Hutterer, K.M.; Polozova, A.; Kuhns, S.; McBride, H.J.; Cao, X.; Liu, J. Assessing analytical and functional similarity of proposed Amgen biosimilar ABP 980 to trastuzumab. BioDrugs 2019 , 33 , 321–333. [ Google Scholar ] [ CrossRef ]
Click here to enlarge figure
Variables | Levels | ||
---|---|---|---|
−1 | 0 | +1 | |
Independent variables | |||
X1 = E-80 (mg) | 30 | 45 | 60 |
X2 = Cholesterol (mg) | 10 | 15 | 20 |
X3 = TPGS (mg) | 5 | 7.5 | 10 |
Dependent variables | Desired response | ||
Y1 = PS (nm) | Minimum | ||
Y2 = PDI | Minimum Maximum | ||
Y3 = ZP (±) |
Independent Variable (Factors) | Dependent Variable (Response) | |||||
---|---|---|---|---|---|---|
Formulation Code | Factor 1 (X1) | Factor 2 (X2) | Factor 3 (X3) | PS (nm) Y1 | PDI Y2 | ZP (±mV) Y3 |
LP1 | 45 | 15 | 7.5 | 152.8 ± 1.54 | 0.375 ± 0.046 | −7.36 |
LP 2 | 60 | 15 | 5 | 189.4 ± 2.06 | 0.423 ± 0.068 | −11.32 |
LP 3 | 30 | 15 | 5 | 135.5 ± 1.36 | 0.355 ± 0.034 | −9.1 |
LP 4 | 30 | 15 | 10 | 143.5 ± 1.48 | 0.362 ± 0.081 | −6.31 |
LP 5 | 30 | 20 | 7.5 | 149.2 ± 1.59 | 0.348 ± 0.059 | −8.26 |
LP 6 | 45 | 20 | 5 | 164.3 ± 1.85 | 0.318 ± 0.047 | −9.69 |
LP 7 | 60 | 15 | 10 | 194.6 ± 2.18 | 0.463 ± 0.065 | −9.2 |
LP 8 | 60 | 10 | 7.5 | 180.6 ± 2.03 | 0.494 ± 0.072 | −10.3 |
LP 9 | 45 | 10 | 5 | 147.9 ± 1.47 | 0.395 ± 0.061 | −8.19 |
LP 10 | 45 | 15 | 7.5 | 152.8 ± 1.54 | 0.375 ± 0.058 | −7.669 |
LP 11 | 30 | 10 | 7.5 | 114.7 ± 1.16 | 0.385 ± 0.055 | −8.88 |
LP 12 | 45 | 10 | 10 | 151.1 ± 1.54 | 0.378 ± 0.053 | −6.8 |
LP 13 | 45 | 15 | 7.5 | 152.8 ± 1.54 | 0.375 ± 0.062 | −7.36 |
LP 14 | 45 | 20 | 10 | 183.6 ± 1.97 | 0.325 ± 0.048 | −9.16 |
LP 15 | 60 | 20 | 7.5 | 198.4 ± 2.38 | 0.338 ± 0.056 | −15.62 |
The statements, opinions and data contained in all publications are solely those of the individual author(s) and contributor(s) and not of MDPI and/or the editor(s). MDPI and/or the editor(s) disclaim responsibility for any injury to people or property resulting from any ideas, methods, instructions or products referred to in the content. |
Share and Cite
Arya, D.K.; Deshpande, H.; Kumar, A.; Chidambaram, K.; Pandey, P.; Anjum, S.; Deepak, P.; Kumar, V.; Kumar, S.; Pandey, G.; et al. HER-2 Receptor and αvβ3 Integrin Dual-Ligand Surface-Functionalized Liposome for Metastatic Breast Cancer Therapy. Pharmaceutics 2024 , 16 , 1128. https://doi.org/10.3390/pharmaceutics16091128
Arya DK, Deshpande H, Kumar A, Chidambaram K, Pandey P, Anjum S, Deepak P, Kumar V, Kumar S, Pandey G, et al. HER-2 Receptor and αvβ3 Integrin Dual-Ligand Surface-Functionalized Liposome for Metastatic Breast Cancer Therapy. Pharmaceutics . 2024; 16(9):1128. https://doi.org/10.3390/pharmaceutics16091128
Arya, Dilip Kumar, Hemali Deshpande, Ashish Kumar, Kumarappan Chidambaram, Prashant Pandey, Shabnam Anjum, Payal Deepak, Vikas Kumar, Santosh Kumar, Giriraj Pandey, and et al. 2024. "HER-2 Receptor and αvβ3 Integrin Dual-Ligand Surface-Functionalized Liposome for Metastatic Breast Cancer Therapy" Pharmaceutics 16, no. 9: 1128. https://doi.org/10.3390/pharmaceutics16091128
Article Metrics
Article access statistics, further information, mdpi initiatives, follow mdpi.
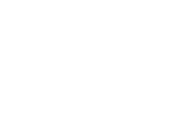
Subscribe to receive issue release notifications and newsletters from MDPI journals
Thank you for visiting nature.com. You are using a browser version with limited support for CSS. To obtain the best experience, we recommend you use a more up to date browser (or turn off compatibility mode in Internet Explorer). In the meantime, to ensure continued support, we are displaying the site without styles and JavaScript.
- View all journals
- Explore content
- About the journal
- Publish with us
- Sign up for alerts
- Review Article
- Open access
- Published: 26 August 2024
The role of cancer-associated fibroblasts and exosomal miRNAs-mediated intercellular communication in the tumor microenvironment and the biology of carcinogenesis: a systematic review
- Reza Nedaeinia ORCID: orcid.org/0000-0001-9922-7181 1 ,
- Simin Najafgholian 2 ,
- Rasoul Salehi 1 , 3 ,
- Mohammad Goli 4 ,
- Maryam Ranjbar 5 ,
- Hamid Nickho 6 ,
- Shaghayegh Haghjooy Javanmard 7 ,
- Gordon A. Ferns 8 &
- Mostafa Manian ORCID: orcid.org/0000-0001-7575-442X 9 , 10
Cell Death Discovery volume 10 , Article number: 380 ( 2024 ) Cite this article
1 Altmetric
Metrics details
- Cancer microenvironment
CAFs (cancer-associated fibroblasts) are highly flexible cells of the cancer microenvironment. They produce the extracellular matrix (ECM) constituents that form the structure of the tumor stroma but are also a source of metabolites, growth factors, chemokines, and exosomes that impact every aspect of the tumor, including its response to treatment. It is believed that exosomal miRNAs facilitate intercellular signaling, which is essential for the development of cancer. The role of miRNAs and CAFs in the tumor microenvironment (TME) and carcinogenesis is reviewed in this paper. The preferred reporting items for systematic reviews and meta-analyses (PRISMA) 2020 guidelines were used to perform a systematic review. Several databases, including Web of Science, Medline, Embase, Cochrane Library, and Scopus, were searched using the following keywords: CAFs, CAF, cancer-associated fibroblasts, stromal fibroblasts, miRNA, exosomal miRNAs, exosome and similar terms. We identified studies investigating exosomal miRNAs and CAFs in the TME and their role in carcinogenesis. A total of 12,572 papers were identified. After removing duplicates ( n = 3803), 8774 articles were screened by title and abstract. Of these, 421 were excluded from further analysis. It has been reported that if exosomal miRNAs in CAFs are not functioning correctly, this may influence the secretory phenotype of tip cells and contribute to increased tumor invasiveness, tumor spread, decreased treatment efficacy, and a poorer prognosis. Under their influence, normal fibroblasts (NFs) are transformed into CAFs. Furthermore, they participate in metabolic reprogramming, which allows for fast proliferation of the cancer cell population, adaptation to growing energy demands, and the capacity to avoid immune system identification.
Similar content being viewed by others
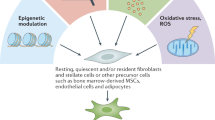
Clinical and therapeutic relevance of cancer-associated fibroblasts
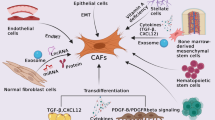
The role of cancer-associated fibroblasts in tumorigenesis of gastric cancer
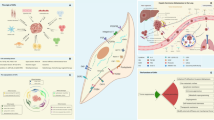
Cancer-associated fibroblasts: overview, progress, challenges, and directions
CAFs interact with immune cells, cancer vasculature, ECM, and tumor cells in the TME to promote the growth of the tumor by secreting a range of cytokines and chemokines
CAFs are dynamic cells that play a crucial role in maintaining tumor structural integrity by producing ECM components and secreting factors that affect tumor growth, invasion, and response to treatment.
The exosomal miRNAs can modulate the behavior of NFs, CAFs, and tumor cells, affecting processes such as conversion from NFs to CAFs, tumor invasiveness, and immune evasion.
It is well recognized that aberrant expression of exosome-derived miRNAs in CAFs contributes significantly to the growth and spread of cancer.
Open questions
Why are CAFs so important in the TME?
What are CAFs in the TME?
What is the role of CAFs and exosomal miRNAs in cancer progression?
Introduction
MicroRNAs are a class of RNAs that are highly conserved and are approximately 25 nucleotides in length; they bind to the 3′ untranslated region (UTR) or open reading frames (ORFs) [ 1 ]. This causes gene silencing after the transcription of target mRNAs. miRNAs regulate gene expression in tumors by utilizing multiple signaling pathways. The miRNAs are important for controlling the interaction between CAFs and tumor cells, which affects how tumors grow and develop. Additionally, they can serve as indicators of cancer and objectives for tumor treatment [ 2 , 3 ].
New insights have emerged concerning the relationship between normal fibroblasts (NFs), CAFs, and cancer cells through exosomal miRNA secretion. Extracellular vesicles can be classified into three primary forms: extracellular vesicles (EVs) are exosomes (~30–150 nm), ectosomes/microvesicles (100–1000 nm), and oncosomes (1–10 µM) [ 4 ]. These types of EVs vary in size and biogenesis. Vesicular trafficking, especially exosome-mediated trafficking, has received attention since each of these three vesicles has a significant function in the biology of cancer [ 5 ]. According to a recent study, the exosome secretion route is regulated by the Rab family proteins, such as 27a and 27b [ 6 ]. It is believed that exosomes facilitate intercellular transmissions, which are essential for cancer growth [ 7 ]. Various cell types in the TME secrete them. They are found in all physiological fluids and are taken up by surrounding cells. Exosomes generated from cancer may operate as intercellular messengers by turning microenvironmental cells into tumor-supportive cells by delivering physiologically active chemicals to these recipient cells [ 8 ].
Exosomal miRNAs can originate from tumor cells or stromal cells such as CAFs [ 9 ]. The majority of research has focused on exosomal miRNAs produced from cancer cells [ 10 ]. Whilst the exosomal miRNAs produced by CAFs are receiving increasing interest, no studies have looked at their abnormal expression patterns in cancer patients. TME includes both the inherent and external variables that influence the development and dissemination of malignancies. It includes the structural, functional, and metabolic aspects of the tumor tissue, as well as the internal environment within the tumor cells. Tumor cells possess the capacity to impact their own survival and growth circumstances through autocrine and paracrine processes, consequently promoting tumor advancement [ 11 ]. The TME and the way that cancer cells interact with their microenvironment depend heavily on CAFs [ 9 ]. An essential part of CAF production and activation is played by malignant cells [ 12 ]. There are several origins of CAFs including 1- bone marrow cells; 2- mesenchymal stem cells (MSCs); 3- ECs (endothelial cells) and pericytes; 4- resident quiescent normal fibroblasts [ 13 ] (Fig. 1 ). Erdogan et al. claim that the direct interactions between tumor cells and other stromal cells, such as immunological and endothelial cells, cause CAFs to establish a certain biological phenotype [ 14 ].
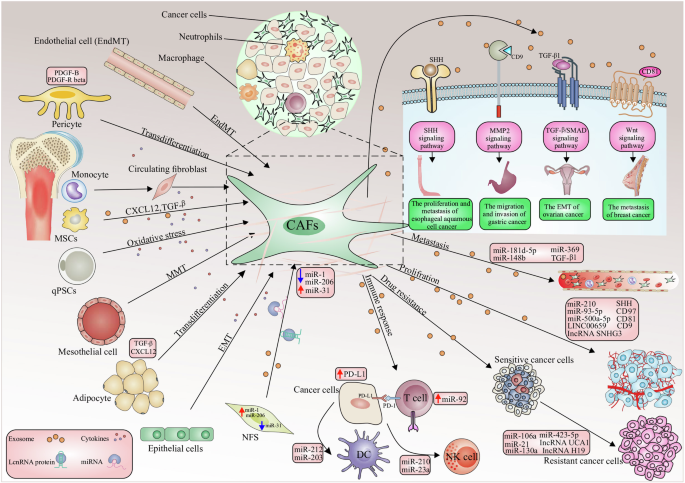
The processes of cell conversion to CAFs consist of the following processes: EMT epithelial-to-mesenchymal transition, EndMT endothelial-to-mesenchymal transition, mesothelial-to-mesenchymal transition MMT. Also, CAF-derived exosomes play a role in the following processes, which include: tumor cell proliferation; conversion of drug-sensitive cancer cells into drug-resistant cancer cells; enhancement of the metastatic capacity of cancer cells; an antitumor immune response by regulating the activity of immune cells. CAF-derived exosomes initiate several molecular processes in tumorigenesis, including TGF-β, to promote EMT, the Wnt signaling cascade contributing to the metastasis of breast cancer, the MMP-2 signaling enhancing the migration and invasiveness of gastric cancer cells. The sonic hedgehog (SHH) signaling pathway thus increases the proliferation and metastasis of ESCC cells.
The TME is comprised of endothelial cells, fibroblasts, pericytes, immune cells, stem cells, and bone marrow precursors, as well as the ECM that surrounds them [ 15 ]. The homeostatic mechanisms that regulate the production and turnover of the ECM, are disturbed in tumors, resulting in the creation of aberrant blood vessels and accumulations of excess fibrillar collagen with a distinct structure [ 16 ]. Disrupted ECM homeostasis results in novel forms of paracrine, cell–cell, and cell–ECM interactions, all of which have significant implications for tumor development, angiogenesis, metastasis, immunosuppression, and treatment resistance [ 15 ]. The TME is crucial for the growth of CAF cells [ 17 ]. Additionally, there is evidence that miRNAs play a significant role in the TME as well as in tumor cells [ 18 ]. The complexity and diversity of the TME are gradually being understood through advancements in science[ 19 ].
The relationship between cancer cells and the TME is regulated by extracellular miRNAs (ex-miRNAs) and miRNAs. MicroRNAs have been shown to regulate the expression of genes that are expressed post-transcriptionally and to be involved in a wide range of normal and abnormal cellular processes [ 20 ]. Recent progress in the assessment of exosomes has made it possible to use miRNAs as biomarkers or therapeutic tools in clinical practice [ 21 ]. Mesenchymal stem cells (MSCs) have the potential to differentiate into CAFs when they interact with miRNAs from tumor exosomes within the TME. CAFs can help with several aspects of tumor development, such as growth, EMT, metabolism, invasion, and metastasis. CAFs have the ability to generate pro-angiogenic factors, which can promote angiogenesis and tumor development [ 22 ]. CAFs are proliferative, metabolically active cells that resemble fibroblasts and can be implicated in any stage of the development of cancer. According to earlier research, CAFs and tumor cells communicate with one another through the secretion of several chemokines and cytokines that are part of the ECM [ 23 ]. Tumor cells can communicate with neighboring cells by secreting soluble molecules into the extracellular space, according to recent research on small extracellular vesicles (sEVs) [ 24 , 25 ]. Exosomes contain a great variety of bioactive molecules, including signal peptides, microRNAs, lipids, and DNA [ 26 ].
Tumor cells release large amounts of exosomes to interact with the environment across extended distances or to deliver paracrine signals [ 27 ]. Therefore, by changing the content of vesicles and transporting chemicals to the tumor site that promote the appearance of oncogenic processes such as proliferation, invasion, proliferation of cancer stem cells, and even treatment resistance, exosomal miRNAs can affect carcinogenesis and cancer progression [ 28 ].
This review focuses on how exosomal miRNAs influence intercellular interaction and are crucial in the way cancer cells interact with their macro- and microenvironment. Exosomes are a constant carrier of miRNAs. Under different circumstances, miRNAs in the plasma exosome can be maintained. The function of exosomal miRNAs and CAFs in the TME and the development of cancer have been reviewed.
The study search method
Our study followed the guidelines of preferred reporting items for systematic reviews (PRISMA) 2020 guidelines for systematic reviews [ 29 ]. To review exosomal miRNAs and CAFs in the TME and carcinogenesis, we searched: Medline, PubMed, Cochrane Library, Embase, Web of Science, and Scopus. We considered studies up until Jan 2023. Additionally, we searched preprint articles on servers like medRxiv and the Social Science Research Network (SSRN). In this search, the following keywords were used: (([Mesh] “MicroRNAs” MicroRNA OR [Handle/Summary] OR miRNAs [Abstract/Title] OR “MicroRNA” [Abstract/Title] miRNA OR [Handle/Summary] OR [Title/Abstract] “Small Temporal RNA” The terms “Tumor-associated fibroblasts” and “Cancer-Associated Fibroblasts” are interchangeable in Mesh. Fibroblasts linked to OR tumors [Title/Abstract] OR “Fibroblasts associated with cancer” [Mesh] OR Fibroblasts associated with cancer [Title/Abstract] OR Fibroblast [Title/Abstract] OR “Fibroblasts” [Mesh] OR “Stromal fibroblasts “[Mesh] Alternatively stated [Title/Abstract])) AND “tumor-associated”[Mesh]“tumor microenvironment “[Mesh] OR Microenvironment *[Introduction/Title] AND (Cancer)[Handle/Summary] OR Carcinogen* [Abstract/Title] Neoplasm OR *[Handle/Summary] Cancer [Handle/Summary] AND (Exosomes*[Title/Abstract] OR (“Exosomes” [Mesh])). To find articles not found by the automated search, a manual search of reference lists and reviews was conducted for potentially eligible articles. We identified duplicate studies and excluded these. We screened the titles and abstracts of the studies and then reviewed the full texts of the articles in two rounds. Two authors, RN and MM, independently studied the two phases. We resolved any disagreements by consulting with RS, the third author.
Search strategy
Study eligibility criteria: inclusion and exclusion.
This review focuses on primary research that examined the relationship between exosomal miRNAs and CAFs in the tumor microenvironment and carcinogenesis. This systematic review was conducted using the following criteria for inclusion: original studies published in either English or Persian that have looked into the role of exosomal miRNAs and CAFs across various types of cancer. Based on the PRISMA- 2020 PICO question formulation checklist, which includes P: Population/Disease/Issue I: Intervention, C: Comparison, O: Outcome, and Text, they will assess the article’s suitability. Our focus was on the TME and carcinogenesis as an outcome, but we also looked at the connection between exosomal miRNAs and CAFs in articles where carcinogenesis was not reported. The current review determined the frequency of exosomal miRNAs and CAFs in cancer patients. Case reports, case series, and studies with less than 6 participants, clinical trials, editorials, commentaries, letters to editors, and narrative reviews were excluded. Additionally, we excluded studies that only looked at exosomal miRNAs and CAFs in specific patient populations, such as those with diabetes or dementia, and studies that involved animals or laboratory settings.
Data extraction
In order to gather articles, eliminate duplicates, and evaluate titles and abstracts, an Endnote library (Version x9) was performed. To begin the process of extracting the data, an expert checklist based on the information gleaned from the articles was created. Only after that was the data extraction carried out. In the studies, information such as the authors’ names, year of publication, country, study design, sample size, age, and gender of patients the study’s specifics, the sample type, the publisher’s nation or area, the detection strategy, and the key findings were collected.
PRISMA flow diagram: study selection process
The study selection process is illustrated in the PRISMA Flow Diagram (Fig. 2 ), outlining the identification, screening, eligibility, and inclusion of studies in this systematic review. 12,572 studies were identified after searching the different databases. 3709 studies were omitted because they were duplicates. The other 8789 articles were checked for eligibility. After evaluating the full texts, 421 studies out of 1109 with titles and abstracts were not included in the analysis (Fig. 2 ). Finally, 77 studies listed in Table 1 were included to perform a systematic review.
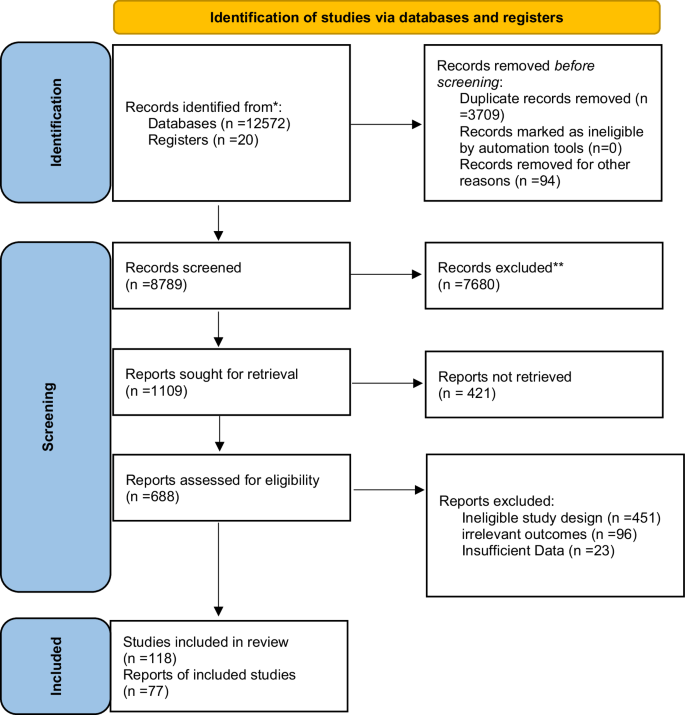
*Consider, if feasible to do so, reporting the number of records identified from each database or register searched (rather than the total number across all databases/registers). **If automation tools were used, indicate how many records were excluded by a human and how many were excluded by automation tools.
Potential role of exosomal miRNAs in the colonization of metastasized CAFs cells
Exosomes and CAFs have important functions in the TME. Exosomes, which are vesicles generated by tumor cells outside of the cells, contain proteins that can interact with immune cells and hinder their capacity to kill tumors, thereby facilitating the evasion of cancer by the immune system. Conversely, CAFs play a crucial role in inhibiting the immune response within the TME, hence promoting tumor invasion and dissemination. Both exosomes and CAFs play a role in creating an immunosuppressive environment in the TME [ 30 ].
Because of the distribution of exosomes to neighboring cells, CAFs play a critical role in regulating the formation of malignancies [ 31 ]. Drug resistance, angiogenesis, invasion, metastasis, and tumor development are all significantly influenced by exosomal miRNAs. They control mechanisms that affect the microenvironment and cancer immunity. Two examples of the endosomal sorting complex required for transport (ESCRT)-containing proteins that are in charge of exosome formation are Alix (Apoptosis-linked gene 2-interacting protein X) and Tsg101 (Tumor susceptibility gene 101 protein). Proteomic investigations of isolated exosomes from various cell types have found Tsg101, Alix, and ubiquitinated proteins [ 32 ].
Recent studies have shown that cancer cells generate more exosomes than normal cells, even in the very early phases of carcinogenesis. Analysis of proteomic profiling of blood-circulating extracellular vesicles in breast cancer holds great promise for early detection and diagnosis [ 33 ]. Tumor-derived exosomes (TEX) are exosomes generated by tumors that are crucial for managing tumor cells inside the TME. It was discovered that TEXs were significant even in the pre-cancer stage. Furthermore, TEXs with physiologically active miRNAs can mirror the unique features of different types of cancer, impacting tumor growth. Induction of the TEM from melanoma cells [ 34 ], transfer of miRNAs supporting 1-integrin-NF-kB signaling from metastatic liver cancer cells [ 35 ], improvement of breast cancer cell motility and ECM remodeling pathways [ 36 ], and activation of SOCS1/JAK2/STAT3 signaling from melanoma cells [ 37 ] (Fig. 1 ). According to a recent study, the presence of miRNAs in TEXs may help tumors survive by changing NFs into CAFs. In human melanoma cells,miR-21, miRNA-211, miR-222, miR-214, miR-155, and miR-210 may enhance the activation of NFs to undergo CAF transformation [ 38 ]. Consequently, there may be a considerable shift in the CAF gene’s expression [ 39 ]. It is thought that miR-21 is the primary regulator of oncogenic processes. It enhances cell survival, CAF formation, and activation by regulating TGF-β1 signaling [ 40 ]. Healthy hepatic stellate cells were converted into CAFs by isolated exosomes from patients with hepatocellular carcinoma (HCC) via miR-21, which downregulated PTEN (Tumor suppressor gene) and activated the PI3K/AKT signaling pathway [ 41 ].
miR-21 has been reported in several types of tumors. The exosomal miR-21-5p released by cancer cells promotes angiogenesis and it is involved in neoplastic processes [ 42 ]. An interesting point is related to the promotion of secretion of angiogenic factors through exosomal miR-21 released from cancer cells and its role in neoplastic processes [ 43 ]. MiR-21 has been shown to activate NFs and produce the CAF phenotypic markers SMA and S100A4, respectively in studies from colorectal and malignant esophageal cancer cells [ 39 ]. The two most common miRNAs, miR-21 and miR-146a, which are recognized as significant regulators of CAF formation, were involved in this process [ 44 , 45 ]. Furthermore, by stimulating the expression of transforming growth factor-β (TGF-β)/ Smad pathway, which can cause endothelial cells (ECs) to convert into CAFs (EMT), exosomal miR-21-5p induces EMT in gastric cancer (GC) [ 46 ] (Fig. 1 ). Next-generation sequencing technology has shown that the exosomal transfer of miR-21 from CAFs to OVCA (Ovarian cancer) cells inhibited apoptosis and increased resistance to treatment with paclitaxel by reducing the expression of apoptotic peptidase activating factor (APAF1) [ 47 ].
When exosomal miR-21-5p causes the peritoneal cavity to undergo mesothelial–mesenchymal transition (MMT), lung cancer is more likely to spread [ 48 ]. Mesothelial cells have been identified as a source of CAFs in peritoneal carcinomatosis (PC) [ 49 ]. Therefore, the exosomal interaction between tumor cells, CAFs, and tumor-associated macrophages (TAMs) is a major factor in the growth of malignancies [ 50 ]. While miR-10b, miR-6819-5p, miR-6737-5p, and miR-1249-5p have been shown to have indirect oncogenic activity via controlling fibroblast function [ 51 , 52 , 53 ]. CAFs release exosomal miR-1228, which has the ability to enhance the invasion and migration of osteosarcoma by targeting suppressor of cancer cell invasion (SCAI). Exosomal collagen type VI alpha 1 (COL6A1), transforms healthy fibroblasts into CAFs, facilitating the invasion and migration of osteosarcoma (OS) cells via the TGF-β/COL6A1 signaling pathway [ 54 , 55 ]. In the context of OS, studies have shown that OS cell-derived exosomal components, such as COL6A1 and leukemia inhibitory factor receptor antisense RNA1 (LIFR-AS1), can induce the transformation of NFs into CAFs. Once activated, these CAFs can enhance the migration and invasion of OS cells through the secretion of proinflammatory cytokines and interaction with microRNAs. Additionally, the interaction between CAF-secreted exosomes and OS cells can promote malignancy grade and contribute to the development of OS. Moreover, CAFs contribute to immune evasion and tumor progression by modulating the immune microenvironment, promoting the differentiation of myofibroblasts, and enhancing angiogenesis [ 55 ]. Furthermore, CAF-derived exosomes have been implicated in promoting angiogenesis within the TME, which is a crucial process for tumor growth. These exosomes can deliver pro-angiogenic factors and signaling molecules to endothelial cells, stimulating the formation of new blood vessels to support the growing tumor. Additionally, CAF-derived exosomes have been associated with immunosuppressive effects, influencing the immune response within the TME and facilitating immune escape by cancer cells [ 54 ].
To what extent can the presence of CAFs affect the development of cancer?
Numerous cell processes, including tumor development, EMT, metabolism, metastasis, and invasion can all be accelerated by CAFs [ 56 ]. The EMT plays an important role in both carcinogenesis and embryogenesis. EMT is mostly responsible for the invasion of single cells, which modifies the morphology and adhesion characteristics of cancer cells and represses epithelial markers in favor of mesenchymal signatures. The expression of genes associated with EMT may be stimulated by the interaction between β-catenin accumulation and T-cell factor/lymphoid enhancer factor (TCF/LEF), a crucial EMT initiator [ 57 ]. A study has demonstrated that the high heterogeneity of CAFs allows them to exhibit two functionalities in disease: while CAFs can promote angiogenesis, inflammation, immunosuppression, and metastasis, which ultimately lead to the growth of tumors, the antitumor characteristics of fibroblasts contribute to processes such as ECM production, the growth and differentiation of epithelial cells, and the response to tissue damage [ 58 ]. CAFs can also produce a variety of growth factors and proinflammatory cytokines, such as TGF-β, vascular endothelial growth factor(VEGF), interleukin-6 (IL-6), and CXC chemokine ligand 12 (CXCL12), to support angiogenesis and attract immunosuppressive cells into the TME to aid in immune evasion [ 59 ]. Furthermore, CAFs can contribute to cancer development indirectly (via soluble factors and miRNA) as well as directly (through intercellular communication). Exosomes generated by CAFs control the TME and mediate the growth and dissemination of cancer cells [ 56 ]. Numerous bioactive substances, including DNA, lipids, signal peptides, and miRNAs, are carried by exosomes. Among these molecules are exosomal miRNAs, which are important regulators of the TME. It is well recognized that aberrant expression of exosome-derived miRNAs in CAFs contributes significantly to the growth and spread of cancer [ 60 ] for example, miR-9 and miR-200s induce NFs in TME to transform into CAFs and promote tumor metastasis [ 36 , 61 ].
CAFs interact with immune cells, cancer vasculature, ECM, and tumor cells in the TME to promote the growth of the tumor by secreting a range of cytokines and chemokines. Proliferative signaling is aided by a number of CAF-derived substances that help cancer cells evade growth suppressors and withstand cell death. Exosomes from the CRC cell lines HT-29 and SW480, perhaps via miRNA let-7d, inhibited the migration of CC-type chemokine receptor 2 (CCR2) monocytes (THP-1 cells) and the release of chemokine (C-C motif) ligand 7 (CCL7) from CAFs in vitro [ 62 ]. According to Fullár et al., membrane-type 1 matrix metalloproteinase (MT1-MMP), which is generated by tumor cells, activates inactive matrix metalloproteinase-2 (MMP-2) produced by fibroblasts. The fine-tuning of cancer cell invasion is dependent on the activated MMP-2 [ 63 ]. In oral squamous cell carcinoma (OSCC) is further increased by the miR-34a-5p/AXL axis. The AKT/GSK3β/β-catenin signaling pathway facilitates this process. This may trigger EMT, which raises the metastasis of cancer cells [ 31 ]. MiR-451 is a tumor suppressor that is regulated in various types of tumors. In contrast, CAFs use small molecules called exosomal miR-451 to signal and help cancer cells move and grow [ 64 ]. Furthermore, it has been shown that the miRNA levels in CAFs and cancer cells may vary differ from those in exosomes [ 9 ]. Tumor stroma has been demonstrated to have a large distribution of CAFs. Myofibroblasts and fibroblasts combine to become CAFs [ 65 ]. It is well known that these CAFs stimulate angiogenesis, which contributes to tumor development and, consequently, to the growth and spread of cancer cells [ 66 ] for example miR-526b and miR-655 promote angiogenesis and lymphangiogenesis in TME [ 67 ].
Recent studies suggest that unregulated epigenetic control of gene expression and metabolic adaptive response may be the cause of the aberrant activation of CAFs [ 68 ]. Regular fibroblasts (NFs) are not like activated CAFs; instead, they change cell surface markers. During carcinogenesis, CAFs’ pro- and antitumorigenic activities are probably dynamic. For example, in order to enhance the CAF phenotype in breast cancer, cancer cells might interact with fibroblasts to stimulate Notch signaling [ 69 ]. However, since squamous cell carcinoma may enhance CAF phenotypes when Notch signaling is absent, this method probably isn’t relevant in all situations [ 70 ]. There is a transfer of gastric cancer (GC)-derived exosomal miR-27a to fibroblasts. This transfection leads to a decrease in cysteine and glycine rich protein 2 (CSRP2) expression, an increase in α-SMA expression, and the separation of fibroblasts into CAFs [ 71 ]. Pancreatic adenocarcinoma (PAAD) remains one of the most common and lethal tumors. Comparing the tissues of PAAD to the corresponding normal tissues, it was shown that the expression of ACTA2, FAP (fibroblast activation protein), PDGFRα/β (platelet-derived growth factor receptor-α/β), and S100A4 (which is widely used as a marker to detect CAFs) was significantly overexpressed [ 72 ]. Numerous inflammatory modulators can activate the CAFs [ 73 ]. Interleukin-1 (IL-1) acts via NF-kB and IL-6, and the transcription factor STAT (signal transducer and activator of transcription) [ 74 ]. Crosstalk and positive feedback mechanisms such as JAK (Janus kinase)-STAT signaling, the contractile cytoskeleton, and modifications in histone acetylation are the root causes of the further increase in CAF activation [ 74 ]. Furthermore, physical alterations occur in the ECM, and CAF is activated [ 75 ]. Therefore, tumor cells are the primary source of CAFs, which accelerates the development of tumors.
The Importance of exosomal miRNAs secreted from CAFs in the spread of cancer and the CAFs
The importance of the microenvironment for the development, maintenance, and spreading of the tumor mass has been mainly shown [ 76 ]. The majority of cell types, including cancer cells, release exosomes, which are nanoparticles with a diameter of 30–150 nm that facilitate communication between surrounding cells and tumors [ 75 ].
Given that miRNAs are crucial regulators of tumor functionalities, it is becoming more and clearer that alterations driven by cancer govern these miRNA-based networks. A member of the α-arrestin protein family is thioredoxin-interacting protein (TXNIP). It is regarded as a thioredoxin-activity endogenous inhibitor. It has been established that TXNIP had a role in the development of colorectal cancer (CRC). Key components in the formation of CRC are TXNIP and miR-135b-5p from CAFs. CAF exosomes may regulate the production of miR-135b-5p to affect CRC cell proliferation by blocking TXNIP [ 18 ].
Unlike other solid tumors, gynecological malignancies have seldom been observed to include exosomes; in particular, endometrial cancer (EC) exosomal miRNAs are thought to play a significant role as a mediator in this two-way communication between cells [ 77 ]. However, exosomal miRNAs act as a bridge for information exchange between TAMs, CAFs, and EC cells. They are crucial for the growth of tumor cells, the EMT transition, and ultimately the development of TMEs. Exosomes generated by CAF stimulate the proliferation of EC. Exosomes produced from EC-CAFs may include downregulated miRNAs with tumor suppressor characteristics. Comparatively to NFs, EC- CAFs contained and released substantially less miR-148b in exosomes. DNA methyltransferase 1 (DNMT1) is suppressed when miR-148b translocates to EC cells. In the event that this suppression is not achieved, EMT will rise and EC will get stronger. Accordingly, the downregulation of miR-148b in EC-CAF-derived exosomes contributes to both EC cell invasion and metastasis. Consequently, treating EC with miR-148b overexpression may be therapeutic [ 78 ]. Similarly, miR-320a expression in EC cells, EC- CAFs-derived exosomes, and EC-CAFs will decline. MiR-320a suppresses the HIF1α/VEGFA axis, which inhibits the proliferation of EC cells [ 79 ]. Poor prognostic indicators such as HIF1α and VEGFA are indicative of EC [ 63 ]. Additionally, there is a link between the rise in EC cell radiation sensitivity and the decline in HIF1α/VEGFA levels [ 80 ]. Exosomes that overexpress miR-320a may also be employed to treat EC sufferers. Studies on serum exosomal miRNAs and miRNAs produced from CAFs in solid tumors [ 81 , 82 ] as well as supraglottic laryngeal squamous cell carcinomas (SLSCC) have also been conducted [ 83 , 84 ]. In patients with SLSCC, exosomal miRNAs generated from CAFs had aberrant expression. Together with their target genes (CCND1, CDKN1B, CDK6, PTEN, and FOS), miR-16-5p, miR-29a-3p, miR-34c-5p, miR-32-5p, and miR-490-5p may form a carcinogenic TME and function as biomarkers for SLSCC therapy [ 10 ] .
There is evidence that in hepatocellular carcinomas (HCCs), exosomes produced from CAF may control the TME. The wnt/β-catenin signaling pathway is decreased by CAF-derived exosomes containing miR-20a-5p oncogene. It causes the inhibition of actin-binding 1 (LIMA1) in HCC and the inhibition of tumor suppressor LIM domain [ 85 ]. GSK3β was effective in β-catenin degradation in prostate cancer (PC). The reason for the growth of PC cells and metastasis through the regulation of GSK3β/β-catenin signaling was actually miR-1290 exosomally secreted CAFs [ 86 ]. Exosomes have a crucial function in facilitating the spread of metastatic castration-resistant prostate cancer (CRPC) by transporting proteins such as APOE, LRG1, and ITIH that are directly implicated in tumor growth and the formation of secondary tumors in the bones. The diagnostic relevance of these exosomal proteins in differentiating between CRPC and PC patients is important, and targeting them could be a feasible strategy for CRPC therapy. Moreover, exosomes discharged by PC cells exhibiting diverse androgen response characteristics can impact the formation of new blood vessels in CRPC, with miR-27a-3p being recognized as a crucial mediator in this mechanism. Gaining a comprehensive understanding of how extracellular vesicles contribute to the spread of metastatic CRPC is essential for identifying and developing novel treatment targets for advanced CRPC [ 87 ].
The reverse transcriptase telomerase, which lengthens telomeres, is one of the characteristics that set cancer cells apart. This is due to the fact that whereas most somatic cells lack the enzyme, the majority of malignant cells possess this enzyme. Through the upregulation of two CAF markers, α-smooth muscle actin (SMA) and vimentin, exosomal telomerase may aid in the conversion of NFs into CAFs. Telomerase activity modifies the miRNA transcriptomes in these fibroblasts. The proliferative phenotype that these cells adopt after eating exosomal human telomerase reverse transcriptase (hTERT) may be influenced by one of the most well-known miRNAs, miR-342, which is thought to be expressed ectopically as a survival benefit [ 88 ]. Moreover, exosomes contain a significant amount of circular RNAs (circRNAs), which have a crucial function in various physiopathological processes including the migration and growth of tumor cells, vascularization in tissues, and the development of tissues and organs. Tumor cells communicate with one another by exchanging exosomal circRNAs, which are signaling molecules, to enhance the growth and progression of tumors. Exosomes have a regulatory impact on tumor formation and change of the tumor microenvironment by carrying circRNAs to tumor cells or other cells in the microenvironment [ 89 ]. Exosomal circRNAs play crucial roles in the TME by modulating cancer hallmarks, including angiogenesis, EMT, invasion, and metastasis. They can also regulate anticancer immunity and enhance chemoresistance [ 89 , 90 ]. Exosomal circRNAs can be used to monitor tumor prognosis and predict postoperative recurrence. For instance, in bladder urethral epithelial carcinoma (UCB), circPRMT5 is overexpressed and positively correlated with low survival in patients. Similarly, a high expression of exosomal circPDE8A is a risk factor for patients with pancreatic ductal adenocarcinoma (PADC), and lower levels of exo-FECR1 are associated with longer disease remissions in small cell lung cancer (SCLC) patients [ 90 ]. In bladder cancer, the expression of CircNFIX in serum exosomes is significantly increased in patients with tumor recurrence compared to those with primary tumors [ 90 ]. As research on exosomal circRNAs progresses, exosomal circRNAs are anticipated to serve as biomarkers for early detection of bladder cancers and as targeted therapy tools [ 89 ]. Exosomal circRNAs have been shown to regulate melanoma progression by modulating the TME. For example, exosomal circRPS5 can inhibit melanoma cell proliferation and invasion [ 91 ]. Recent studies have shown that exosomal circRNAs play critical roles in conferring chemotherapy resistance in diverse cancers. Exosomal circRNAs can act as miRNA sponges, interact with RNA-binding proteins, and even encode proteins, thereby modulating the expression of genes involved in drug resistance pathways [ 92 ]. In these cancers (lung cancer, gynecological cancers, glioma, breast cancer, prostate cancer, multiple myeloma, and oral squamous cell carcinoma), specific exosomal circRNAs were found to be upregulated and associated with increased proliferation, migration, invasion, and apoptosis resistance in chemotherapy-resistant cancer cells. Mechanistic investigations revealed that these exosomal circRNAs typically regulate chemoresistance by sponging miRNAs and altering the expression of target genes [ 92 ].
The role of CAFs in the resistance to anticancer drugs
Exosomal miRNAs originating from cancer can promote CAF differentiation, and exosomal miRNAs released by CAFs in the TME are essential in the development of therapeutic resistance. Tumor-derived exosomes may have a major impact on the differentiation of CAFs, which increases tumor development, invasive, pro-angiogenic, and drug-resistant phenotypes [ 34 , 93 ]. Furthermore, Cancer’s response to chemotherapy is controlled by CFAs. Premetastatic lung fibroblasts were similarly stimulated by exosomal miR-1247-3p from HCC cells, which resulted in the overexpression of IL1B, IL-6, and IL-8 as well as resistance to sorafenib therapy [ 35 ]. Similarly, exosomal miR-1247-3p from HCC cells triggered CAF in fibroblasts of a lung premetastatic niche. Proinflammatory genes such as IL1B, IL-6, and IL-8 were upregulated as a result, and sorafenib therapy led to the development of therapeutic resistance [ 35 ]. Sarfenib is regarded as a targeted therapy for the treatment of advanced HCC, despite its modest effect in terms of overall survival because of drug resistance [ 94 ]. In terms of clinical prognosis, sorafenib is unsuccessful due to inflammatory interleukins such as IL-6 [ 94 ].
Diverse subtypes of CAFs coexist in pancreatic cancer tissues, where they have the dual ability to accelerate and impede the disease’s progression. Here, cancer cells with p53 mutations that cause GOF (gain of function) give rise to the prevailing population known as CAFs [ 95 ]. As a result, it gives p53-null and GOF cancer cells a metastatic environment [ 95 ]. GOF mutant p53 cells or their CAFs have the ability to rewire CAFs that were trained by null p53 cancer cells. One important component of this pro-metastatic milieu is perlecan (Heparin sulfate proteoglycan 2, or HSPG2). These dominating CAFs impede the capacity of cancer cells to respond to chemotherapy. Because decreasing perlecan in the stroma in conjunction with chemotherapy boosts mice survival, perlecan is a possible target for anti-stromal treatments in pancreatic cancer. CAFs have the ability to release exosomes carrying miR-20a, which can speed up the development and chemoresistance of non-small-cell lung cancer (NSCLC) [ 96 ]. CAF-derived exosomes, especially those that are CD9-positive, inhibit the growth of malignant melanoma cells. The five-year disease-free survival rate was considerably greater in patients who had CD9-positive CAF-made exosomes compared to patients who lacked CD9 exosomes [ 97 ]. Tumors with high levels of periostin-positive (POSTN) CAFs significantly decreased the overall survival of the patients [ 98 ]. POSTN+CAF showed minimal expression of smooth muscle actin and high rates of protein synthesis and proliferation when they were found in peri-/pre-tumoral regions. They were associated with highly malignant tumors and macrophage infiltrates. The recruitment of dendritic cells and immune-related markers was associated with CAFs that were podoplanin-positive. Specific TME traits were associated with the simultaneous presence of POSTN + CAF and podoplanin-positive CAFs, as measured by stromal abundance and immune cell infiltrates. Although the published myofibroblastic CAF (myCAF)/iCAF categorization was unrelated to POSTN + CAF, podoplanin-positive CAFs showed a fraction that resembled the inflammatory CAF (iCAF). According to these findings, a POSTN + CAF is an early, activated CAF that is associated with aggressive malignancies, whereas a podoplanin-positive CAF is connected to an immune-related phenotype. Together, these two categories establish specific TME and influence patient prognosis; they might be useful for future patient stratification. Targeting or reprogramming “bad” CAF populations (e.g., POSTN) may lead to the development of a novel treatment strategy for pancreatic ductal adenocarcinoma (PDAC) [ 98 ]. The platinum-based medication cisplatin creates adducts in the DNA of cancer cells to exert its anticancer effects. These adducts drive cancer cells to undergo apoptosis, or cell death, by inducing the DNA damage response. Cisplatin resistance is a major issue for people with bladder cancer (BC). It has been demonstrated that the delivery of bioactive compounds by exosomes derived from CAFs (CAF-Exo) can enhance chemotherapy resistance in a range of human tumors. Exosomal LINC00355, generated from CAFs, enhances BC cell resistance to cisplatin by modulating the miR-34b-5p/ABCB1 axis [ 99 ]. A recent study found that CAF-Exo miR-98-5p downregulates CDKN1A, a crucial regulator of cell cycle arrest, increasing OVCA cell proliferation and fostering a cisplatin-resistant phenotype [ 100 ]. Interestingly, several exosomal miRNAs from CAF have been shown to enhance cisplatin resistance. For example, in head and neck cancer cells, CAF-Exo miR-196a reduced the expression of CDKN1B (Cyclin Dependent Kinase Inhibitor 1B), a gene critical for the change from the G1 to the S phases of the cell cycle [ 101 ]. Furthermore, miR-522, which was produced from CAF exosomes, conferred cisplatin resistance to gastric cancer cells [ 102 ]. In the pancreatic TME, CAF-Exo miR-106b was found to directly reduce tumor protein 53-induced nuclear protein 1 (TP53INP1) expression, which in turn increased gemcitabine resistance in pancreatic cancer cells [ 12 ]. In patients with PAAD receiving gemcitabine therapy, exosomal miR-146a, which is released by CAF, has also been shown to target Snail pathways and accelerate the establishment of the gemcitabine-resistant phenotype [ 103 ]. Nano-drug delivery systems (nano-DDSs) can be designed to respond to TME stimuli, enhancing drug retention, accumulation, penetration, and tumor cell uptake [ 104 ]. Exosomes have emerged as a promising drug delivery platform for cancer therapies, including melanoma treatment [ 91 , 105 ]. Exosomes can be engineered to enhance their tumor-targeting capabilities and drug delivery efficiency. Strategies include surface decoration and loading exosomes with therapeutic cargoes [ 91 , 105 ]. Engineered exosomes can deliver drugs to tumor sites and temporally release therapeutic molecules, improving on the limitations of conventional molecular-targeted drugs [ 91 ]. Moreover, nanomaterials used in tumor immunotherapy are into two main categories: organic and inorganic nanomaterials, organic nanomaterials include polymers such as polylactic–co-glycolic acid (PLGA), polyethyleneimine (PEI), polyethylene glycol (PEG), polycaprolactone (PCL), and polyglutamic acid ( γ -PGA), as well as cell membrane-derived structures like tumor cell membranes, macrophage membranes, platelet membranes, and erythrocyte membranes. These organic nanomaterials exhibit advantageous properties like biocompatibility but may face challenges such as instability and rapid metabolism. In contrast, inorganic nanomaterials like graphene, black phosphorus, and silicon demonstrate enhanced stability and drug delivery capabilities but potentially pose greater toxicity concerns [ 106 ]. Lili Cheng et al. designed and created a therapeutic nanovesicle known as hGLV. Cheng et al. developed a hybrid therapeutic nanovesicle, named hGLV, by combining genetically modified exosomes with drug-containing thermosensitive liposomes. The overexpression of CD47 in hGLV was seen to modify the process of tumor cell phagocytosis by macrophages through the inhibition of CD47 signaling [ 107 ] Nanoparticle-enhanced radiotherapy is significant in triggering robust cancer immunotherapy because it allows for the combination of antitumor drugs to kill tumors while also enhancing the body’s immunity. This multi-treatment effect is achieved through the use of multifunctional nanomaterials, which can overcome the limitations of traditional immunotherapy strategies such as low bioavailability, low response rates, and severe side effects. By utilizing nanotechnology, researchers have been able to break through the bottleneck problem of antitumor immunotherapy, offering new possibilities for more effective cancer treatment [ 108 ].
The CAFs within the TME interact with cancer cells in ways that are essential to the growth and dissemination of disease. It is now recognized that within the TME, CAFs, and myofibroblasts are extremely diverse cells with unique gene expression patterns and a variety of biological roles that often contradict one another [ 72 ] (Fig. 1 ). Multiple CAF subpopulations may be seen inside a single tumor. CAFs partly mediate their actions by altering the ECM and secreting soluble components and EVs. EVs, of which exosomes are a subclass, are microscopic sacs that contain a range of biomolecules, such as proteins, nucleic acids, and lipids. Exosomes transport a payload of lipids, proteins, and nucleic acids that resemble the biological makeup of the cells from which they were derived. The tumor’s malignant nature is regulated by its surroundings. The TME is composed of biological components such as tumor cells, CAFs, endothelium, and immunological cells, as well as non-cellular components such as exosomes and cytokines, which all play important roles [ 109 ]. CAFs are stromal cells within the TME that have been found to promote tumor growth, invasion, and metastasis. They play a significant role in creating a supportive environment for cancer cells by secreting various factors that aid in tumor progression. In particular, CAF-derived exosomes have been implicated in mediating communication between CAFs and cancer cells, thereby promoting tumor progression and therapy resistance. These exosomes may contain specific bioactive molecules that contribute to the crosstalk within the TME, ultimately impacting tumor growth and response to therapy [ 92 ]. CAF secretes proinflammatory cytokines such as IL-1 and IL-8, both of which have pro-tumor effects. According to studies, tumor-derived exosome miRNA-1247-3p can activate tumor-associated fibroblasts, causing them to produce cytokines such as IL-6 and IL-8, which promotes lung metastasis of liver cancer [ 35 ]. Numerous investigations have revealed that exosome-associated miRNA-9 and miRNA-200s enhance metastasis and the transformation of NFs into CAFs [ 36 ]. Multiple vascular supports are required for cancer progression and can provide nourishment and growth factors to tumors. Exosomes released in low oxygen conditions augment the stem cell characteristics in Ewing’s sarcoma by delivering a concentrated amount of miR-210 that suppresses the apoptotic pathway, resulting in cell viability and promoting the creation of cellular spheres. In the microenvironment of bone sarcomas, EVs have a vital function in facilitating communication between different cells. Additionally, they can be used as biomarkers to aid in the diagnosis and prognosis of these conditions. An analysis is conducted on the role of EVs in facilitating communication between various cells inside the microenvironment of bone sarcomas. This research yields novel insights that can aid in the identification of therapeutic targets and diagnostic analysis [ 110 ].
Previous studies have demonstrated that exosomal miRNAs released by tumor cells have a significant influence on vasculature remodeling via IL-8-activated VCAM-1. Exosome-associated miRNA-526b and miRNA-655 also increase lymphangiogenesis and angiogenesis [ 111 ], whereas miRNA-340-5p and miRNA-561 promote an immunosuppressive TME [ 112 , 113 ]. Exosomes produced from CAFs have been demonstrated to greatly promote OVCA tumor growth. CAFs directly transported exosomes into OVCA cells to boost intracellular circIFNGR2 levels. Exosomal circIFNGR2 activation inhibited cell proliferation, metastasis, and EMT. Mechanistically, elevated circIFNGR2 activated the miR-378/ST5 axis, preventing tumor cells from evolving into malignant cells [ 109 ]. The methods by which CAFs influence PC tumorigenesis remain unknown. When CAFs-Exo was compared to NFs-Exo, the level of miR-1290 was considerably greater. CAFs dramatically improved PC cell motility, invasion, stemness, and metastasis by transmitting exosomal miR-1290 [ 114 ]. The lack of precise CAF markers substantially limits depletion techniques. Nonetheless, CAF depletion was the first technique to be shown as a supplement to immunotherapies. Reducing FAzP (fibroblast activation protein) + CAFs enhanced the anticancer vaccine’s efficacy, according to seminal research by Kraman et al. [ 115 ]. Recent research has identified CAF-mediated CXCL12 expression as a defining characteristic of the “CAF-S1” immunosuppressive subtype of breast cancer myofibroblastic CAF (myCAF) [ 116 ]. Pre-clinical studies have also shown that the clinically approved inhibitor AMD3100 is effective in blocking the interaction between CXCL12 and its cognate receptor CXCR4 [ 117 , 118 ]. The complex and intricate signaling network that involves TGF-β, PI3Ks/AKT/mTOR (Mammalian target of rapamycin), MAPK (Mitogen-activated protein kinase), Wnt, Janus kinase/signal transducers and activators of transcription, EGFR (Epidermal growth factor receptor), Hippo, and nuclear factor kappa-light-chain-enhancer of activated B cells, among other signaling pathways, makes CAFs vulnerable to crosstalk with cancer cells. Exosomes carrying miRNA are released by CAFs, healthy fibroblasts, and cancer cells that form a network of cell-to-cell communication in the TME. These CAF signals reveal distinct characteristics as the disease advances and may be the target of anticancer therapy.
CAFs are essential elements of the TME and play an important role in the interaction between cancer cells and their environment. An essential function of cancer cells is to mediate the development and activation of CAFs. Furthermore, the development of cancer is dependent on both CAF activation and malignant cancer cells. Oncological behaviors are caused by crosstalk between cancer cells and CAFs. Within the TME, NFs, cancer cells, and CAFs all release miRNA-containing exosomes that facilitate cell-to-cell communication. Consequently, CAFs require a focused treatment that improves anticancer therapy in both in vivo and in vitro investigations. MiRNAs are involved in cancer cells as well as the tumor microenvironment. According to the body of data, the relationship between tumor cells and the TME may be impacted by the dysregulation of miRNAs and ex-miRNAs. Thus, it is anticipated that removing CAFs will make it easier to cure cancer and prevent cancer cells from metastasizing and developing resistance. However, a major barrier to more readily treating cancer with therapeutic techniques is the absence of precise markers for CAFs.
Javanmard SH, Vaseghi G, Ghasemi A, Rafiee L, Ferns GA, Esfahani HN, et al. Therapeutic inhibition of microRNA-21 (miR-21) using locked-nucleic acid (LNA)-anti-miR and its effects on the biological behaviors of melanoma cancer cells in preclinical studies. Cancer cell Int. 2020;20:384. https://doi.org/10.1186/s12935-020-01394-6
Article PubMed PubMed Central CAS Google Scholar
Zhang T, Zhang P, Li H-XJ. CAFs-derived exosomal miRNA-130a confers cisplatin resistance of NSCLC cells through PUM2-dependent packaging. Int J Nanomed. 2021:561–77.
Yang S-S, Ma S, Dou H, Liu F, Zhang S-Y, Jiang C. et al. Breast cancer-derived exosomes regulate cell invasion and metastasis in breast cancer via miR-146a to activate cancer associated fibroblasts in tumor microenvironment. Exp Cell Res. 2020;391:111983
Article PubMed CAS Google Scholar
Kumar A, Kumar P, Sharma M, Kim S, Singh S, Kridel SJ, et al. Role of extracellular vesicles secretion in paclitaxel resistance of prostate cancer cells. Cancer Drug Resist. 2022;5:612–24. https://doi.org/10.20517/cdr.2022.26
Lu Y, Eguchi T, Sogawa C, Taha EA, Tran MT, Nara T, et al. Exosome-based molecular transfer activity of macrophage-like cells involves viability of oral carcinoma cells: size exclusion chromatography and concentration filter method. Cells. 2021;10:1328.
Jaé N, McEwan DG, Manavski Y, Boon RA, Dimmeler S. Rab7a and Rab27b control secretion of endothelial microRNA through extracellular vesicles. FEBS Lett. 2015;589:3182–8.
Article PubMed Google Scholar
Albino D, Falcione M, Uboldi V, Temilola DO, Sandrini G, Merulla J, et al. Circulating extracellular vesicles release oncogenic miR-424 in experimental models and patients with aggressive prostate cancer. Commun Biol. 2021;4:119. https://doi.org/10.1038/s42003-020-01642-5
Maacha S, Bhat AA, Jimenez L, Raza A, Haris M, Uddin S, et al. Extracellular vesicles-mediated intercellular communication: roles in the tumor microenvironment and anti-cancer drug resistance. Mol Cancer. 2019;18:55. https://doi.org/10.1186/s12943-019-0965-7
Article PubMed PubMed Central Google Scholar
Yang F, Ning Z, Ma L, Liu W, Shao C, Shu Y, et al. Exosomal miRNAs and miRNA dysregulation in cancer-associated fibroblasts. Mol Cancer. 2017;16:148. https://doi.org/10.1186/s12943-017-0718-4
Wu C, Wang M, Huang Q, Guo Y, Gong H, Hu C, et al. Aberrant expression profiles and bioinformatic analysis of CAF‐derived exosomal miRNAs from three moderately differentiated supraglottic LSCC patients. J Clin Lab Anal. 2022;36:e24108.
Xu M, Li S. The opportunities and challenges of using PD-1/PD-L1 inhibitors for leukemia treatment. Cancer Lett. 2024;593:216969. https://doi.org/10.1016/j.canlet.2024.216969
Fang Y, Zhou W, Rong Y, Kuang T, Xu X, Wu W, et al. Exosomal miRNA-106b from cancer-associated fibroblast promotes gemcitabine resistance in pancreatic cancer. Exp Cell. Res. 2019;383:111543.
Akkız H. Emerging role of cancer-associated fibroblasts in progression and treatment of hepatocellular carcinoma. Int J Mol Sci. 2023;24:3941.
Erdogan B, Ao M, White LM, Means AL, Brewer BM, Yang L, et al. Cancer-associated fibroblasts promote directional cancer cell migration by aligning fibronectin. J Cell Biol. 2017;216:3799–816. https://doi.org/10.1083/jcb.201704053
Li Y, Gu L. Establishment and characterization of HXWMF-1: the first mouse fibroblastic tumor cell line derived from leukemia-associated fibroblasts. Cancer Cell Int. 2021;21:177. https://doi.org/10.1186/s12935-021-01870-7
Sun B. The mechanics of fibrillar collagen extracellular matrix. Cell Rep Phys Sci. 2021;2. https://doi.org/10.1016/j.xcrp.2021.100515
Mao X, Xu J, Wang W, Liang C, Hua J, Liu J, et al. Crosstalk between cancer-associated fibroblasts and immune cells in the tumor microenvironment: new findings and future perspectives. Mol Cancer. 2021;20:131. https://doi.org/10.1186/s12943-021-01428-1
Yin H, Yu S, Xie Y, Dai X, Dong M, Sheng C, et al. Cancer-associated fibroblasts-derived exosomes upregulate microRNA-135b-5p to promote colorectal cancer cell growth and angiogenesis by inhibiting thioredoxin-interacting protein. Cell Signal. 2021;84:110029.
Kang Y, Li S. Nanomaterials: Breaking through the bottleneck of tumor immunotherapy. Int J Biol Macromol. 2023;230:123159. https://doi.org/10.1016/j.ijbiomac.2023.123159
Wu H-J, Hao M, Yeo SK, Guan J-L. FAK signaling in cancer-associated fibroblasts promotes breast cancer cell migration and metastasis by exosomal miRNAs-mediated intercellular communication. Oncogene. 2020;39:2539–49.
Wang J-W, Wu X-F, Gu X-J, Jiang X-H. Exosomal miR-1228 from cancer-associated fibroblasts promotes cell migration and invasion of osteosarcoma by directly targeting SCAI. Oncol Res. 2019;27:979.
You X, Wu J, Zhao X, Jiang X, Tao W, Chen Z, et al. Fibroblastic galectin-1-fostered invasion and metastasis are mediated by TGF-β1-induced epithelial-mesenchymal transition in gastric cancer. Aging. 2021;13:18464.
Ene–Obong A, Clear AJ, Watt J, Wang J, Fatah R, Riches JC, et al. Activated pancreatic stellate cells sequester CD8 + T cells to reduce their infiltration of the juxtatumoral compartment of pancreatic ductal adenocarcinoma. Gastroenterology 2013;145:1121–32.
de la Fuente A, Alonso-Alconada L, Costa C, Cueva J, Garcia-Caballero T, Lopez-Lopez R, et al. M-Trap: exosome-based capture of tumor cells as a new technology in peritoneal metastasis. J Natl Cancer Inst. 2015;107. https://doi.org/10.1093/jnci/djv184
Jeon B-H, Jang C, Han J, Kataru RP, Piao L, Jung K, et al. Profound but dysfunctional lymphangiogenesis via vascular endothelial growth factor ligands from CD11b+ macrophages in advanced ovarian cancer. Cancer Res. 2008;68:1100–9.
Nedaeinia R, Manian M, Jazayeri M, Ranjbar M, Salehi R, Sharifi M. et al. Circulating exosomes and exosomal microRNAs as biomarkers in gastrointestinal cancer. Cancer Gene Ther. 2017;24:48–56.
Whiteside TL. Tumor-derived exosomes and their role in cancer progression. Adv Clin Chem.2016;74:103–41.
Saravanan PB, Kalivarathan J, Khan F, Shah R, Levy MF, Kanak MA. Exosomes in transplantation: role in allograft rejection, diagnostic biomarker, and therapeutic potential. Life Sci. 2023;324:121722. https://doi.org/10.1016/j.lfs.2023.121722
Page MJ, McKenzie JE, Bossuyt PM, Boutron I, Hoffmann TC, Mulrow CD, et al. The PRISMA 2020 statement: an updated guideline for reporting systematic reviews. BMJ. 2021;372:n71. https://doi.org/10.1136/bmj.n71/J
Zhu X, Li S. Nanomaterials in tumor immunotherapy: new strategies and challenges. Mol Cancer. 2023;22:94. https://doi.org/10.1186/s12943-023-01797-9
Li Y-Y, Tao Y-W, Gao S, Li P, Zheng J-M, Zhang S-E, et al. Cancer-associated fibroblasts contribute to oral cancer cells proliferation and metastasis via exosome-mediated paracrine miR-34a-5p. EBioMedicine 2018;36:209–20.
Colombo M, Moita C, van Niel G, Kowal J, Vigneron J, Benaroch P. et al. Analysis of ESCRT functions in exosome biogenesis, composition and secretion highlights the heterogeneity of extracellular vesicles. J Cell Sci. 2013;126:5553–65. https://doi.org/10.1242/jcs.128868 .
Rontogianni S, Synadaki E, Li B, Liefaard MC, Lips EH, Wesseling J, et al. Proteomic profiling of extracellular vesicles allows for human breast cancer subtyping. Commun Biol. 2019;2:325. https://doi.org/10.1038/s42003-019-0570-8
Yeon JH, Jeong HE, Seo H, Cho S, Kim K, Na D, et al. Cancer-derived exosomes trigger endothelial to mesenchymal transition followed by the induction of cancer-associated fibroblasts. Acta Biomater. 2018;76:146–53.
Fang T, Lv H, Lv G, Li T, Wang C, Han Q, et al. Tumor-derived exosomal miR-1247-3p induces cancer-associated fibroblast activation to foster lung metastasis of liver cancer. Nat Commun. 2018;9:191.
Baroni S, Romero-Cordoba S, Plantamura I, Dugo M, D’ippolito E, Cataldo A, et al. Exosome-mediated delivery of miR-9 induces cancer-associated fibroblast-like properties in human breast fibroblasts. Cell Death Dis. 2016;7:e2312–e.
Zhou X, Yan T, Huang C, Xu Z, Wang L, Jiang E, et al. Melanoma cell-secreted exosomal miR-155-5p induce proangiogenic switch of cancer-associated fibroblasts via SOCS1/JAK2/STAT3 signaling pathway. J Exp Clin Cancer Res. 2018;37:1–15.
Article Google Scholar
Jorge NA, Cruz JG, Pretti MAM, Bonamino MH, Possik PA, Boroni M. Poor clinical outcome in metastatic melanoma is associated with a microRNA-modulated immunosuppressive tumor microenvironment. J Transl Med. 2020;18:1–17.
Shu SL, Yang Y, Allen CL, Maguire O, Minderman H, Sen A, et al. Metabolic reprogramming of stromal fibroblasts by melanoma exosome microRNA favours a pre-metastatic microenvironment. Sci Rep. 2018;8:12905.
Li Q, Zhang D, Wang Y, Sun P, Hou X, Larner J, et al. MiR-21/Smad 7 signaling determines TGF-β1-induced CAF formation. Sci Rep. 2013;3:1–9.
Google Scholar
Zhou Y, Ren H, Dai B, Li J, Shang L, Huang J, et al. Hepatocellular carcinoma-derived exosomal miRNA-21 contributes to tumor progression by converting hepatocyte stellate cells to cancer-associated fibroblasts. J Exp Clin Cancer Res. 2018;37:1–18.
He Q, Ye A, Ye W, Liao X, Qin G, Xu Y, et al. Cancer-secreted exosomal miR-21-5p induces angiogenesis and vascular permeability by targeting KRIT1. Cell Death Dis. 2021;12:576. https://doi.org/10.1038/s41419-021-03803-8
Wu D, Qin H, Wang Z, Yu M, Liu Z, Peng H, et al. Bone mesenchymal stem cell-derived sEV-encapsulated thermosensitive hydrogels accelerate osteogenesis and angiogenesis by release of exosomal miR-21. Front Bioeng Biotechnol. 2022;9:829136.
Kumarswamy R, Volkmann I, Jazbutyte V, Dangwal S, Park D-H, Thum T. Transforming growth factor-β–induced endothelial-to-mesenchymal transition is partly mediated by microRNA-21. Arterioscler Thromb Vasc Biol. 2012;32:361–9.
Hsieh J-Y, Huang T-S, Cheng S-M, Lin W-S, Tsai T-N, Lee OK, et al. miR-146a-5p circuitry uncouples cell proliferation and migration, but not differentiation, in human mesenchymal stem cells. Nucleic Acids Res. 2013;41:9753–63.
Li Q, Li B, Li Q, Wei S, He Z, Huang X, et al. Exosomal miR-21-5p derived from gastric cancer promotes peritoneal metastasis via mesothelial-to-mesenchymal transition. Cell Death Dis. 2018;9:854.
Au Yeung CL, Co N-N, Tsuruga T, Yeung T-L, Kwan S-Y, Leung CS, et al. Exosomal transfer of stroma-derived miR21 confers paclitaxel resistance in ovarian cancer cells through targeting APAF1. Nat Commun. 2016;7:11150.
Watabe S, Kikuchi Y, Morita S, Komura D, Numakura S, Kumagai‐Togashi A, et al. Clinicopathological significance of microRNA‐21 in extracellular vesicles of pleural lavage fluid of lung adenocarcinoma and its functions inducing the mesothelial to mesenchymal transition. Cancer. Medicine. 2020;9:2879–90.
CAS Google Scholar
Lee J, Hong BS, Ryu HS, Lee H-B, Lee M, Park IA, et al. Transition into inflammatory cancer-associated adipocytes in breast cancer microenvironment requires microRNA regulatory mechanism. PLoS ONE. 2017;12:e0174126.
Li M, He L, Zhu J. Targeting tumor-associated macrophages for cancer treatment. Cell Biosci. 2022;12:85. https://doi.org/10.1186/s13578-022-00823-5 .
Yoshii S, Hayashi Y, Iijima H, Inoue T, Kimura K, Sakatani A, et al. Exosomal microRNAs derived from colon cancer cells promote tumor progression by suppressing fibroblast TP 53 expression. Cancer Sci. 2019;110:2396–407.
Li H, Li F. Exosomes from BM-MSCs increase the population of CSCs via transfer of miR-142-3p. Br J Cancer. 2018;119:744–55.
Dai G, Yao X, Zhang Y, Gu J, Geng Y, Xue F, et al. Colorectal cancer cell–derived exosomes containing miR-10b regulate fibroblast cells via the PI3K/Akt pathway. Bull Cancer. 2018;105:336–49.
Yue J, Chen ZS, Xu XX, Li S. Functions and therapeutic potentials of exosomes in osteosarcoma. Acta Mater Med. 2022;1:552–62. https://doi.org/10.15212/amm-2022-0024
Gao X, Gao B, Li S. Extracellular vesicles: a new diagnostic biomarker and targeted drug in osteosarcoma. 2022;13. https://doi.org/10.3389/fimmu.2022.1002742
Hu J, Wang W, Lan X, Zeng Z, Liang Y, Yan Y, et al. CAFs secreted exosomes promote metastasis and chemotherapy resistance by enhancing cell stemness and epithelial-mesenchymal transition in colorectal cancer. Mol Cancer. 2019;18:1–15.
Medici D, Hay ED, Olsen BR. Snail and Slug promote epithelial-mesenchymal transition through β-catenin–T-cell factor-4-dependent expression of transforming growth factor-β3. Mol Biol Cell. 2008;19:4875–87.
Monteran L, Erez N. The dark side of fibroblasts: cancer-associated fibroblasts as mediators of immunosuppression in the tumor microenvironment. Front Immunol. 2019;10:1835. https://doi.org/10.3389/fimmu.2019.01835
Ahmadzadeh M, Rosenberg SA. TGF-β1 attenuates the acquisition and expression of effector function by tumor antigen-specific human memory CD8 T cells. J Immunol. 2005;174:5215–23.
Minciacchi VR, Spinelli C, Reis-Sobreiro M, Cavallini L, You S, Zandian M, et al. MYC mediates large oncosome-induced fibroblast reprogramming in prostate cancer. Cancer Res. 2017;77:2306–17.
Tang X, Hou Y, Yang G, Wang X, Tang S, Du Y. et al. Stromal miR-200s contribute to breast cancer cell invasion through CAF activation and ECM remodeling. Cell Death Differ. 2016;23:132–45.
Noh GT, Kwon J, Kim J, Park M, Choi D-W, Cho K-A, et al. Verification of the role of exosomal microRNA in colorectal tumorigenesis using human colorectal cancer cell lines. PLoS ONE. 2020;15:e0242057.
Fullár A, Kovalszky I, Bitsche M, Romani A, Schartinger VH, Sprinzl GM, et al. Tumor cell and carcinoma-associated fibroblast interaction regulates matrix metalloproteinases and their inhibitors in oral squamous cell carcinoma. Exp Cell Res. 2012;318:1517–27.
Khazaei S, Nouraee N, Moradi A, Mowla SJC, Oncology T. A novel signaling role for miR-451 in esophageal tumor microenvironment and its contribution to tumor progression. ClinTransl Oncol. 2017;19:633–40.
Orimo A, Gupta PB, Sgroi DC, Arenzana-Seisdedos F, Delaunay T, Naeem R, et al. Stromal fibroblasts present in invasive human breast carcinomas promote tumor growth and angiogenesis through elevated SDF-1/CXCL12 secretion. Cell. 2005;121:335–48.
Erez N, Truitt M, Olson P, Hanahan D. Cancer-associated fibroblasts are activated in incipient neoplasia to orchestrate tumor-promoting inflammation in an NF-κB-dependent manner. Cancer Cell. 2010;17:135–47.
Hunter S, Nault B, Ugwuagbo K, Maiti S, Majumder M. Mir526b and Mir655 promote tumour associated angiogenesis and lymphangiogenesis in breast cancer. Cancers. 2019;11. https://doi.org/10.3390/cancers11070938
Lee YT, Tan YJ, Falasca M, Oon CE. Cancer-associated fibroblasts: epigenetic regulation and therapeutic intervention in breast cancer. Cancers. 2020;12. https://doi.org/10.3390/cancers12102949
Strell C, Paulsson J, Jin S-B, Tobin NP, Mezheyeuski A, Roswall P. et al. Impact of epithelial-stromal interactions on peritumoral fibroblasts in ductal carcinoma in situ. J Natl Cancer Inst. 2019;111:983–95.
Procopio M-G, Laszlo C, Al Labban D, Kim DE, Bordignon P, Jo S-H, et al. Combined CSL and p53 downregulation promotes cancer-associated fibroblast activation. Nat Cell Biol. 2015;17:1193–204.
Wang J, Guan X, Zhang Y, Ge S, Zhang L, Li H. et al. Exosomal miR-27a derived from gastric cancer cells regulates the transformation of fibroblasts into cancer-associated fibroblasts. Cell Physiol Biochem. 2018;49:869–83.
Sugimoto H, Mundel TM, Kieran MW, Kalluri R. Identification of fibroblast heterogeneity in the tumor microenvironment. Cancer Biol Ther. 2006;5:1640–6.
Sahai E, Astsaturov I, Cukierman E, DeNardo DG, Egeblad M, Evans RM. et al. A framework for advancing our understanding of cancer-associated fibroblasts. Nat Rev Cancer. 2020;20:174–86.
Albrengues J, Bertero T, Grasset E, Bonan S, Maiel M, Bourget I, et al. Epigenetic switch drives the conversion of fibroblasts into proinvasive cancer-associated fibroblasts. Nat Commun. 2015;6:10204.
Calvo F, Ranftl R, Hooper S, Farrugia AJ, Moeendarbary E, Bruckbauer A, et al. Cdc42EP3/BORG2 and septin network enables mechano-transduction and the emergence of cancer-associated fibroblasts. Cell Rep. 2015;13:2699–714.
Calvo F, Ege N, Grande-Garcia A, Hooper S, Jenkins RP, Chaudhry SI. et al. Mechanotransduction and YAP-dependent matrix remodelling is required for the generation and maintenance of cancer-associated fibroblasts. Cell report. 2013;15:637–46.
Sykaras AG, Christofidis K, Politi E, Theocharis S. Exosomes on endometrial cancer: a biomarkers treasure trove?. Cancer. 2022;14:1733
Article CAS Google Scholar
Li BL, Lu W, Qu JJ, Ye L, Du GQ, Wan XP. Loss of exosomal miR-148b from cancer-associated fibroblasts promotes endometrial cancer cell invasion and cancer metastasis. J Cell Physiol. 2019;234:2943–53. https://doi.org/10.1002/jcp.27111
Zhang N, Wang Y, Liu H, Shen W. Extracellular vesicle encapsulated microRNA-320a inhibits endometrial cancer by suppression of the HIF1α/VEGFA axis. Exp Cell Res. 2020;394:112113.
Miyasaka A, Oda K, Ikeda Y, Sone K, Fukuda T, Inaba K, et al. PI3K/mTOR pathway inhibition overcomes radioresistance via suppression of the HIF1-α/VEGF pathway in endometrial cancer. Gynecol Oncol. 2015;138:174–80. https://doi.org/10.1016/j.ygyno.2015.04.015
Shan G, Zhou X, Gu J, Zhou D, Cheng W, Wu H, et al. Downregulated exosomal microRNA-148b-3p in cancer associated fibroblasts enhance chemosensitivity of bladder cancer cells by downregulating the Wnt/β-catenin pathway and upregulating PTEN. Cell Oncol. 2021;44:45–59.
Liu Y, Yang Y, Du J, Lin D, Li F. MiR‐3613‐3p from carcinoma‐associated fibroblasts exosomes promoted breast cancer cell proliferation and metastasis by regulating SOCS2 expression. IUBMB Life. 2020;72:1705–14.
Zhao Q, Zheng X, Guo H, Xue X, Zhang Y, Niu M, et al. Serum exosomal miR-941 as a promising oncogenic biomarker for laryngeal squamous cell carcinoma. J Cancer. 2020;11:5329.
Wang J, Zhou Y, Lu J, Sun Y, Xiao H, Liu M, et al. Combined detection of serum exosomal miR-21 and HOTAIR as diagnostic and prognostic biomarkers for laryngeal squamous cell carcinoma. Med Oncol. 2014;31:1–8.
Qi Y, Wang H, Zhang Q, Liu Z, Wang T, Wu Z, et al. CAF-released exosomal miR-20a-5p facilitates HCC progression via the LIMA1-mediated β-catenin pathway. Cells. 2022;11:3857.
Wang S, Du P, Cao Y, Ma J, Yang X, Yu Z. et al.Cancer associated fibroblasts secreted exosomal miR-1290 contributes to prostate cancer cell growth and metastasis via targeting GSK3β. Cell Death Discov. 2022;8:371
Li S, Kang Y, Zeng Y. Targeting tumor and bone microenvironment: Novel therapeutic opportunities for castration-resistant prostate cancer patients with bone metastasis. Biochim Biophys Acta Rev Cancer. 2024;1879:189033. https://doi.org/10.1016/j.bbcan.2023.189033
Likonen D, Pinchasi M, Beery E, Sarsor Z, Signorini LF, Gervits A, et al. Exosomal telomerase transcripts reprogram the microRNA transcriptome profile of fibroblasts and partially contribute to CAF formation. Sci Rep. 2022;12:16415.
Liu Q, Li S. Exosomal circRNAs: novel biomarkers and therapeutic targets for urinary tumors. Cancer Lett. 2024;588:216759. https://doi.org/10.1016/j.canlet.2024.216759
Xu Y, Kong S, Qin S, Shen X, Ju S. Exosomal circRNAs: sorting mechanisms, roles and clinical applications in tumors. Front Cell Dev Biol. 2020;8:581558. https://doi.org/10.3389/fcell.2020.581558
Xu M, Li S. Nano-drug delivery system targeting tumor microenvironment: a prospective strategy for melanoma treatment. Cancer Lett. 2023;574:216397. https://doi.org/10.1016/j.canlet.2023.216397
Guo X, Gao C, Yang DH, Li S. Exosomal circular RNAs: a chief culprit in cancer chemotherapy resistance. Drug Resist Update. 2023;67:100937. https://doi.org/10.1016/j.drup.2023.100937
Ringuette Goulet C, Bernard G, Tremblay S, Chabaud S, Bolduc S, Pouliot F. Exosomes induce fibroblast differentiation into cancer-associated fibroblasts through TGFβ signaling. Mol Cancer Res. 2018;16:1196–204.
Lai S-C, Su Y-T, Chi C-C, Kuo Y-C, Lee K-F, Wu Y-C, et al. DNMT3b/OCT4 expression confers sorafenib resistance and poor prognosis of hepatocellular carcinoma through IL-6/STAT3 regulation. J Exp Clin Cancer Res. 2019;38:1–18.
Vennin C, Mélénec P, Rouet R, Nobis M, Cazet AS, Murphy KJ, et al. CAF hierarchy driven by pancreatic cancer cell p53-status creates a pro-metastatic and chemoresistant environment via perlecan. Nat Commun. 2019;10:3637.
Shi L, Zhu W, Huang Y, Zhuo L, Wang S, Chen S, et al. Cancer‐associated fibroblast‐derived exosomal microRNA‐20a suppresses the PTEN/PI3K‐AKT pathway to promote the progression and chemoresistance of non‐small cell lung cancer. Clin Transl Med. 2022;12:e989.
Fujii N, Yashiro M, Hatano T, Fujikawa H, Motomura H. CD9-positive exosomes derived from cancer-associated fibroblasts might inhibit the proliferation of malignant melanoma cells. Anticancer Res. 2023;43:25–33. https://doi.org/10.21873/anticanres.16130
Neuzillet C, Nicolle R, Raffenne J, Tijeras-Raballand A, Brunel A, Astorgues-Xerri L, et al. Periostin- and podoplanin-positive cancer-associated fibroblast subtypes cooperate to shape the inflamed tumor microenvironment in aggressive pancreatic adenocarcinoma. J Pathol. 2022;258:408–25. https://doi.org/10.1002/path.6011
Luo G, Zhang Y, Wu Z, Zhang L, Liang C, Chen X. Exosomal LINC00355 derived from cancer-associated fibroblasts promotes bladder cancer cell resistance to cisplatin by regulating miR-34b-5p/ABCB1 axis. Acta Biochim Biophys Sin. 2021;53:558–66.
Guo H, Ha C, Dong H, Yang Z, Ma Y, Ding Y. Cancer-associated fibroblast-derived exosomal microRNA-98-5p promotes cisplatin resistance in ovarian cancer by targeting CDKN1A. Cancer Cell Int. 2019;19:1–15.
Qin X, Guo H, Wang X, Zhu X, Yan M, Wang X, et al. Exosomal miR-196a derived from cancer-associated fibroblasts confers cisplatin resistance in head and neck cancer through targeting CDKN1B and ING5. Genome Biol. 2019;20:1–21.
Zhang H, Deng T, Liu R, Ning T, Yang H, Liu D, et al. CAF secreted miR-522 suppresses ferroptosis and promotes acquired chemo-resistance in gastric cancer. Mol Cancer. 2020;19:1–17.
Richards KE, Zeleniak AE, Fishel ML, Wu J, Littlepage LE, Hill R. Cancer-associated fibroblast exosomes regulate survival and proliferation of pancreatic cancer cells. Oncogene. 2017;36:1770–8.
Zhu H, Zhang P, Shi J, Kou D, Bai X. Exosome-delivered circRPS5 inhibits the progression of melanoma via regulating the miR-151a/NPTX1 axis. PLoS ONE. 2023;18:e0287347. https://doi.org/10.1371/journal.pone.0287347
Zhang M, Hu S, Liu L, Dang P, Liu Y, Sun Z, et al. Engineered exosomes from different sources for cancer-targeted therapy. Signal Transduct Target Ther. 2023;8:124 https://doi.org/10.1038/s41392-023-01382-y
Chen Z, Yue Z, Yang K, Shen C, Cheng Z, Zhou X, et al. Four ounces can move a thousand pounds: the enormous value of nanomaterials in tumor immunotherapy. 2023;12:2300882. https://doi.org/10.1002/adhm.202300882 .
Cheng L, Zhang X, Tang J, Lv Q, Liu J. Gene-engineered exosomes-thermosensitive liposomes hybrid nanovesicles by the blockade of CD47 signal for combined photothermal therapy and cancer immunotherapy. Biomater. 2021;275:120964.
Chen Z, Yue Z, Yang K, Li S. Nanomaterials: small particles show huge possibilities for cancer immunotherapy. J Banobiotechnol. 2022;20:484. https://doi.org/10.1186/s12951-022-01692-3
Chen X, Ren X, E J, Zhou Y, Bian R Exosome-transmitted circ IFNGR2 Modulates Ovarian Cancer Metastasis via miR-378/ST5 Axis. Molecular and cellular biology. 2023:1-21.
Li S. The basic characteristics of extracellular vesicles and their potential application in bone sarcomas. J Nanobiotechnol. 2021;19:277. https://doi.org/10.1186/s12951-021-01028-7
Hunter S, Nault B, Ugwuagbo KC, Maiti S, Majumder M. Mir526b and Mir655 promote tumour associated angiogenesis and lymphangiogenesis in breast cancer. Cancers. 2019;11:938.
Chen E-B, Zhou Z-J, Xiao K, Zhu G-Q, Yang Y, Wang B, et al. The miR-561-5p/CX3CL1 signaling axis regulates pulmonary metastasis in hepatocellular carcinoma involving CX3CR1+ natural killer cells infiltration. Theranostics. 2019;9:4779.
Liu Y, Li X, Zhang Y, Wang H, Rong X, Peng J, et al. An miR-340-5p-macrophage feedback loop modulates the progression and tumor microenvironment of glioblastoma multiforme. Oncogene. 2019;38:7399–415.
Wang S, Du P, Cao Y, Ma J, Yang X, Yu Z, et al. Cancer associated fibroblasts secreted exosomal miR-1290 contributes to prostate cancer cell growth and metastasis via targeting GSK3&beta. Cell Death Discov. 2022;8:371.
Kraman M, Bambrough PJ, Arnold JN, Roberts EW, Magiera L, Jones JO, et al. Suppression of antitumor immunity by stromal cells expressing fibroblast activation protein–α. Science. 2010;330:827–30.
Costa A, Kieffer Y, Scholer-Dahirel A, Pelon F, Bourachot B, Cardon M, et al. Fibroblast heterogeneity and immunosuppressive environment in human breast cancer. Cancer Cell. 2018;33:463–79.e10.
Feig C, Jones JO, Kraman M, Wells RJ, Deonarine A, Chan DS, et al. Targeting CXCL12 from FAP-expressing carcinoma-associated fibroblasts synergizes with anti–PD-L1 immunotherapy in pancreatic cancer. Proc Natl Acad Sci USA. 2013;110:20212–7.
Chen IX, Chauhan VP, Posada J, Ng MR, Wu MW, Adstamongkonkul P, et al. Blocking CXCR4 alleviates desmoplasia, increases T-lymphocyte infiltration, and improves immunotherapy in metastatic breast cancer. Proc Natl Acad Sci USA. 2019;116:4558–66.
Download references
This work was supported by the Vice Chancellery for Research, Isfahan University of Medical Science, Isfahan, Iran (No. 140033).
Author information
Authors and affiliations.
Pediatric Inherited Diseases Research Center, Research Institute for Primordial Prevention of Non-Communicable Disease, Isfahan University of Medical Sciences, Isfahan, Iran
Reza Nedaeinia & Rasoul Salehi
Department of Emergency Medicine, School of Medicine, Valiasr Hospital, Arak University of Medical Sciences, Arak, Iran
Simin Najafgholian
Department of Genetics and Molecular Biology, School of Medicine, Isfahan University of Medical Sciences, Isfahan, Iran
Rasoul Salehi
Department of Food Science and Technology, Laser and Biophotonics in Biotechnologies Research Center, Isfahan (Khorasgan) Branch, Islamic Azad University, Isfahan, Iran
Mohammad Goli
Advanced Materials Research Center, Department of Materials Engineering, Najafabad Branch, Islamic Azad University, Najafabad, Iran
Maryam Ranjbar
Department of Immunology, Faculty of Medicine, Iran University of Medical Sciences, Tehran, Iran
Hamid Nickho
Applied Physiology Research Center, Cardiovascular Research Institute, Isfahan University of Medical Sciences, Isfahan, Iran
Shaghayegh Haghjooy Javanmard
Brighton and Sussex Medical School, Division of Medical Education, Falmer, Brighton, Sussex, UK
Gordon A. Ferns
Department of Medical Laboratory Science, Faculty of Medical Science Kermanshah Branch, Islamic Azad University, Kermanshah, Iran
Mostafa Manian
Isfahan Neurosciences Research Center, Isfahan University of Medical Sciences, Isfahan, Iran
You can also search for this author in PubMed Google Scholar
Contributions
RN and MM designed research. RS, MR, MG, SHJ, SN, and HN were involved in the search strategy and writing original draft preparation. RN wrote the paper. GAF revised the manuscript critically for important intellectual content. All authors read and approved the final manuscript.
Corresponding authors
Correspondence to Reza Nedaeinia or Mostafa Manian .
Ethics declarations
Competing interests.
The authors declare no competing interests.
Additional information
Publisher’s note Springer Nature remains neutral with regard to jurisdictional claims in published maps and institutional affiliations.
Rights and permissions
Open Access This article is licensed under a Creative Commons Attribution 4.0 International License, which permits use, sharing, adaptation, distribution and reproduction in any medium or format, as long as you give appropriate credit to the original author(s) and the source, provide a link to the Creative Commons licence, and indicate if changes were made. The images or other third party material in this article are included in the article’s Creative Commons licence, unless indicated otherwise in a credit line to the material. If material is not included in the article’s Creative Commons licence and your intended use is not permitted by statutory regulation or exceeds the permitted use, you will need to obtain permission directly from the copyright holder. To view a copy of this licence, visit http://creativecommons.org/licenses/by/4.0/ .
Reprints and permissions
About this article
Cite this article.
Nedaeinia, R., Najafgholian, S., Salehi, R. et al. The role of cancer-associated fibroblasts and exosomal miRNAs-mediated intercellular communication in the tumor microenvironment and the biology of carcinogenesis: a systematic review. Cell Death Discov. 10 , 380 (2024). https://doi.org/10.1038/s41420-024-02146-5
Download citation
Received : 08 April 2024
Revised : 24 July 2024
Accepted : 12 August 2024
Published : 26 August 2024
DOI : https://doi.org/10.1038/s41420-024-02146-5
Share this article
Anyone you share the following link with will be able to read this content:
Sorry, a shareable link is not currently available for this article.
Provided by the Springer Nature SharedIt content-sharing initiative
Quick links
- Explore articles by subject
- Guide to authors
- Editorial policies


IMAGES
COMMENTS
Interpreting research (9) Presenting research findings There are two basic ways that data can be presented: quantitatively or qualitatively. Quantitative data is in a numerical form, so it can be can be counted and then presented through
Planning for research. 1 .Selecting a research focus. 2. Selecting appropriate sampling methods. 3. Proposing how research will be conducted. 4, Creating a timeline for research goals. Progress update: All classes completed Week 1&2. Progress update: In progress (to complete rough plan by Week 2)
HSC CAFS Notes: Victoria Fryer [email protected] Home Syllabus Core 1 Core 2 ... Research Fundamentals - The purpose of research, eg advance knowledge, increase understanding, educate others, inform practice ... Presenting research findings - Analysing research results
Presenting research findings . A sociogram is: A map that is used to track/ record interactions between individuals within a group; directional arrows show the flow of interaction. ... The interactions of our CAFS class! Your friendship group or another group in Year 11. Your family.
Once I had this I pieced together the different parts of the question to form my question. Managing resources, eg time, materials. Conducting research. Accessing sources of data. Collecting and recording data. Documenting actions and issues. Interpreting research. Presenting research findings. Analysing research results.
observe the specific roles various individuals adopt within groups, and present research findings. ... CAFS Independent Research Project (IRP). Community and Family Studies- Unit 4 95% (274) 13. CAFS - Prelim Notes Families And Communities.
Learn about: Observations as a Primary Research Method: Conducting and recording observations Advantages and Disadvantages Presenting research findings Learn to: Observe the specific roles various individuals adopt within groups and present findings Requires the observer to watch and record the behaviours of their subjects.
HSC CAFS - Research Methodology - Research Process. Flashcards. Learn. Test. Match. Flashcards. Learn. Test. Match. Created by. SharmainJade. Terms in this set (11) ... Presenting Research Findings Analysis Research Results Drawing Conclusions. Presenting Research Findings. Quantitative Data - graphs, tables and statistics
Presenting Research Findings There are two basic ways that data can be presented - Quantitative or Qualitative. Quantitative data is in a numerical form, so it can be can be counted and then presented through the use of bar, line or pie graphs; or tables with statistics or percentages.
Presenting research findings, e.g. tables, graphs, compiling results Analysing research results, e.g. comparing secondary and primary data ... CAFS: People with disabilities. 46 terms. Feker_Yibeltal. CAFS TERM 3 - SOCIAL IMPACT OF TECHNOLOGY - YELLOW. 64 terms. courteneyturner1. CAFS Core 1- Ethical Behaviour. 6 terms. indiabarnhill. Sets ...
The authors also provide insights and perspectives on future research and clinical studies involving CAFs. ... of antigen-presenting CAFs ... these findings indicate that αSMA + CAFs, namely ...
4. Proposing suitable Research Methods and Sampling Size 5. Developing the Topic Plan CONDUCTING RESEARCH 6. Accessing Sources of Data 7. Collecting and Recording Data 8. Documenting Actions and Issues INTERPRETING RESEARCH 9. Presenting Research Findings 10. Analysing Research Results 11. Drawing Conclusions from Research 12.
Purpose of Review This review describes the presentation, diagnosis, and management of congenital coronary artery fistulas (CAFs) in adults. Recent Findings CAFs are classified as coronary-cameral or coronary arteriovenous fistulas. Fistulous connections at the distal coronary bed are more likely to be aneurysmal with higher risk of thrombosis and myocardial infarction (MI). Medium-to-large or ...
PFM, CACD, IPAD - Planning for research - Formulating a research proposal - Managing resources, e.g time and money - Conducting research - Accessing sources of data-Collecting and recording data - Documenting Actions and issues - Interpreting research - Presenting research findings-Analysing Research results - Drawing conclusions from research
In recent years, multiple studies have drawn attention to the regulatory role of CAFs in tumor metastasis, with findings indicating that CAF infiltration correlates with metastatic propensity through specific protein immunohistochemical staining [13].Coculture experiments with CAFs have demonstrated enhanced tumor cell proliferation, invasion, and metastatic phenotypes, underscoring the ...
- recording actions and proposing solutions to any research issues - presenting primary data in graphs, tables or written reports - comparing key findings from primary and secondary data - forming research-based conclusions and making recommendations - crediting sources of data by means of bibliography and appendix
Schauer et al. presented findings indicating that a high level of IL-1β in ovarian cancer stimulates IL-1R1 on adjacent CAFs, leading to the inhibition of P53 and transactivation of the NF-κB ...
These studies demonstrate that some CAF subpopulations have tumor suppressor effects to some extent. On the basis of current findings, CAFs can be described as a group of cells with functional heterogeneity (Fig. 2). Research and work are urgently entailed to elucidate the clinical relevance of CAF heterogeneity.
Despite centuries since the discovery and study of cancer, cancer is still a lethal and intractable health issue worldwide. Cancer-associated fibroblasts (CAFs) have gained much attention as a pivotal component of the tumor microenvironment. The versatility and sophisticated mechanisms of CAFs in facilitating cancer progression have been elucidated extensively, including promoting cancer ...
These findings are in accordance with various studies that describe the existence of CAF subpopulations in the TME with the ability to present antigens, termed antigen-presenting CAFs (apCAFs ...
documenting actions and issues. after then collecting the findings, the research needs to be interpreted, and that could be through presenting research findings, analysing research results and drawing conclusions from research. 2. explain the importance of confidentiality when conducting research into sensitive topics 8 marks
Hence, these findings suggest that in situ MHCII antigen presentation within the tumor environment by a specialized subset of CAFs contributes to tumor immunity. Author information Authors and ...
Building on the understanding of cancer antigen presentation by CAFs and the second touch hypothesis, which postulates that full T cell polarization requires interaction with antigen-presenting ...
In recent years, biologists and clinicians have witnessed prominent advances in in vitro 3D culture techniques related to biomimetic human/animal tissue analogs. Numerous data have confirmed that unicellular and multicellular (tumoroids) tumor spheroids with dense native cells in certain matrices are sensitive and valid analytical tools for drug screening, cancer cell dynamic growth, behavior ...
Human epidermal growth factor receptor-2 (HER2)-positive breast cancer metastasis remains the primary cause of mortality among women globally. Targeted therapies have revolutionized treatment efficacy, with Trastuzumab (Trast), a monoclonal antibody, targeting HER2-positive advanced breast cancer. The tumor-homing peptide iRGD enhances the intratumoral accumulation and penetration of ...
According to earlier research, CAFs and tumor cells communicate with one ... According to these findings, ... Delaunay T, Naeem R, et al. Stromal fibroblasts present in invasive human breast ...